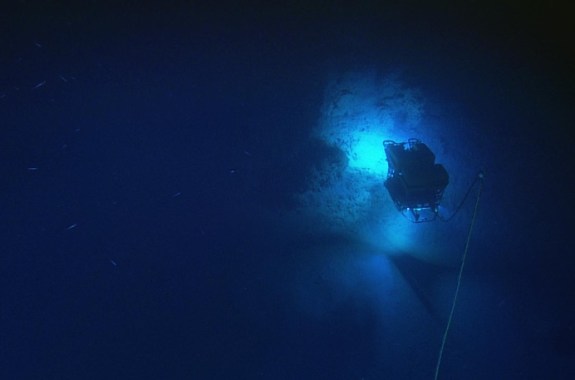

High Pressure in the Deep Ocean
Grade level, 15 min - 1 hr, physical science, activity type:, data analysis , pressure experiments , pressure demonstrations , ocean exploration theme, pressure is a challenge to deep sea explorers.
Though oceans cover more than 70 percent of Earth’s surface, less than 5 percent of our oceans have been explored. Research and commercial vessels travel the surface of the ocean regularly, but the deepest parts of the ocean remain largely unseen by humans. The ocean floor in particular is home to unique marine organisms, as well as enormous geological features like volcanoes, canyons, and ridgelines. In order to take full advantage of these opportunities for discovery, scientists and explorers must first overcome one of the biggest challenges of deep ocean exploration: extreme pressure.
Pressure in the Deep
Everything in the deep ocean is under a great deal of pressure. At any depth in the ocean, the weight of the water above pushes on any object below it. With every foot an object descends into the ocean, more water is pushing down and against it, and more pressure is exerted upon that object. In fact, for every 10 meters traveled deeper into the ocean, there are an additional 6.47kg (14.27lbs) of pressure on each square inch of surface. In order to descend to greater ocean depths, scientists and explorers must use specially designed equipment like remotely operated vehicles (ROVs) and manned submersibles that can operate under extreme pressures. How extreme? An ROV diving to a depth of 2,000m experiences over 1,270kg (2,800lbs) of force exerted on each square inch of its surface!
Remembering Nereus, Explorer of Ocean Depths
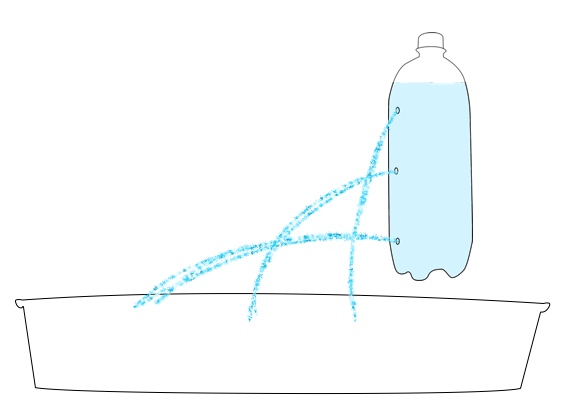
Visualize the Pressure-Depth Relationship
You can observe the effects of this increase of pressure with depth in this classroom activity. All you will need is a soda bottle, electrician’s tape, and a sink.
Click through this slideshow to learn how.
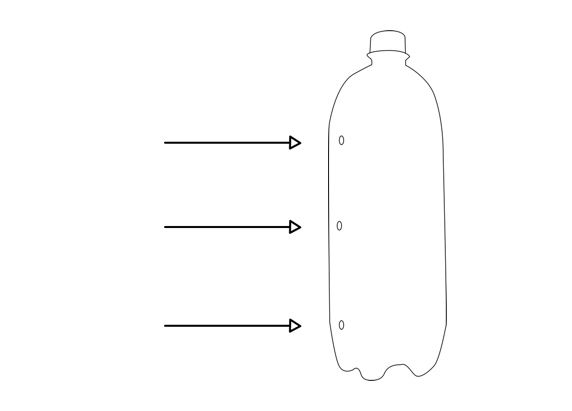
Make three evenly spaced holes down the side of a soda bottle. This demonstration works best when the holes are circular and about one pencil-width in diameter. For best results, have an adult use a drill or soldering iron to make the holes.
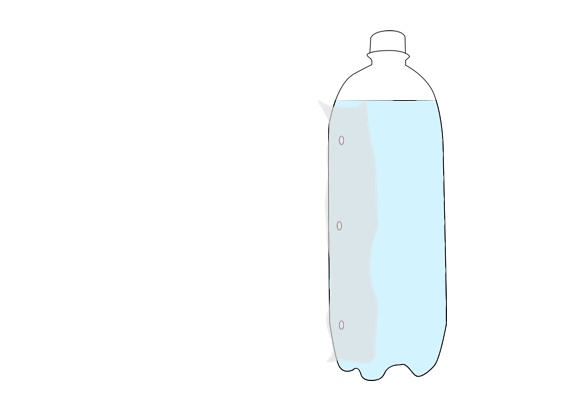
Cover all of the holes with a single, long strip of tape, or get a student to cover them with their fingers. Fill the bottle with water.
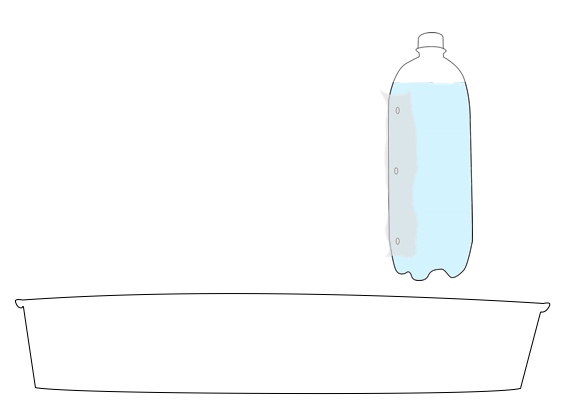
Hold the bottle upright over a large bowl, in a sink, or over a surface you don’t mind getting wet. Make sure the bottle is raised above the edge of the dish so all the holes are visible.
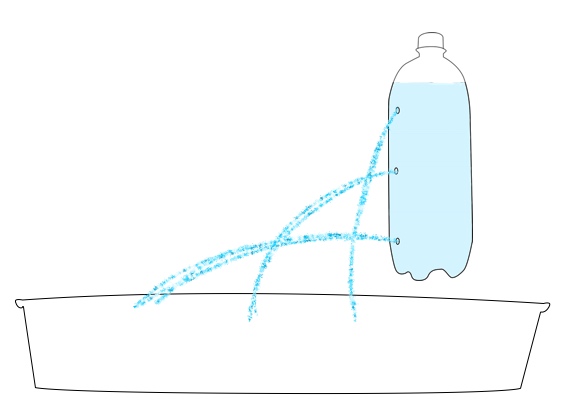
Quickly uncover all of the holes at once, and watch what happens. The water will shoot out of the holes at different distances due to the different water pressure at each hole. Water from the hole closest to the bottle’s neck will travel the shortest distance, while water from the bottom hole, where pressure is highest, will travel the farthest.
Compressible Foam
A favorite pastime aboard exploration and research vessels like the exploration vessel (E/V) Nautilus is decorating foam cups to place atop ROVs during exploratory dives into the deep ocean (the authors of this educational resource traveled aboard the E/V Nautilus in 2015 as Science Communication Fellows). Aboard the E/V Nautilus , members of the Corps of Exploration stuff each foam cup with paper towels to help them keep their shape, then put the cups in a mesh bag tied to a milk crate attached to the ROV Argus that plunges into the ocean. After the exploratory dive is complete, the ROV is brought back up to the deck of the ship, and the cups are removed. Instead of returning to the surface the same size they went down, the cups are dramatically smaller, but weigh nearly the same amount. Why do the foam cups shrink?
Foam cups are made from expanded polystyrene foam (EPF). Like most foam, EPF is made up of tiny polystyrene bubbles stuck together. When a foam cup descends into the ocean attached to an ROV, the air that fills its tiny polystyrene bubbles is compressed, and the polystyrene bubbles are crushed. At the bottom of the ocean, the pressure from the water is so great in all directions that the polystyrene beads are compressed uniformly, and the foam cups shrink to a fraction of their original size. At a depth of 2,000m, the pressure on a foam cup is equivalent to the weight of about 500 fully-grown giant Galápagos tortoises pressing down on it, so they stand no chance!
While compressing foam cups is fun for sea explorers, for fish and ROVs, compressibility is something to be avoided! The ROVs aboard the E/V Nautilus emerge from the depths the same size they went down because they are specially-designed using materials like aluminum, PVC, steel, and glass that are much, much less compressible than foam. Meanwhile, fish that travel to the deep ocean have specially-adapted bodies that are filled mostly with incompressible fluids instead of air, so that as they descend, their bodies retain their shape and size.
Simulate the Pressure Conditions of the Deep Ocean
Want to try your hand at pressure compaction at home? You can replicate the pressures of the deep ocean on EPF using a pump or syringe.
Safety note: Because of the high pressure generated in this activity, there is a risk that the bottle used may burst or detach from the pump. All observers should wear safety goggles and stay 4-6 feet from the demonstration. For added safety, have one person hold the bottle while a second uses the pump to add pressure.
2L soda bottle
2.5 cups polystyrene beads (or small marshmallows in a pinch)
Paper funnel
Bike pump or large plastic needleless syringe (>50 milliliter)
Duct tape or electrician’s tape
Permanent maker
Safety glasses
Water rocket pop-bottle connector, or other connector to form an airtight seal between the bottle and the pump. To download directions on how to make your own using a bike inner tube, bottle cap, and drill, click here: How to make a bike pump soda bottle adapter .
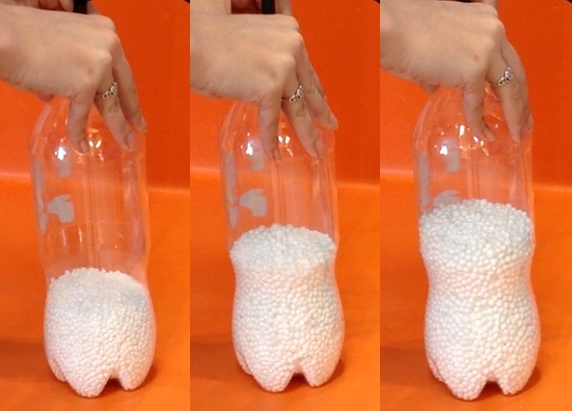
How to Simulate the Pressure Conditions of the Deep Ocean
Want to try your hand at pressure compaction at home? You can replicate the pressures of the deep ocean on EPF at home using a pump, soda bottle, and some foam beads.
Click through the slideshow to learn more.
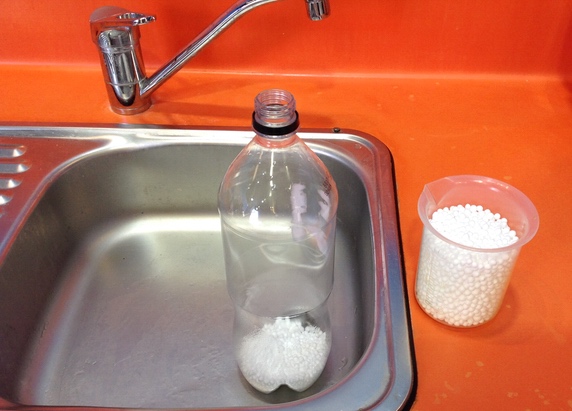
In an enclosed space like a sink or bathtub, carefully pour polystyrene beads into the bottle, using a paper funnel if needed. Using a permanent marker, draw a line on the bottle to show the starting volume of the beads.
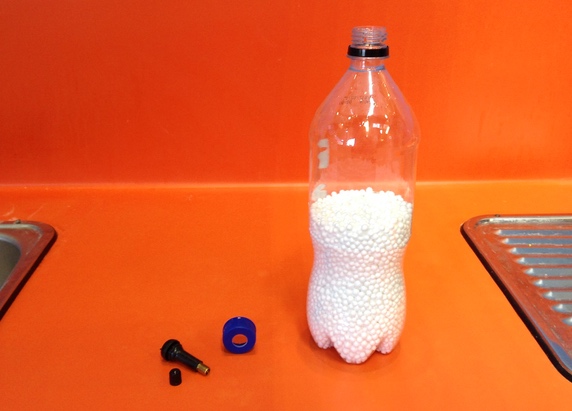
Fit a water rocket connector or cork to the neck of the bottle. Next, attach the connector or cork to the pump, and hold the connection firmly. For added safety, wrap electrical tape or duct tape tightly around the bottleneck, bike pump, and adapter to prevent detachment or air leakage.
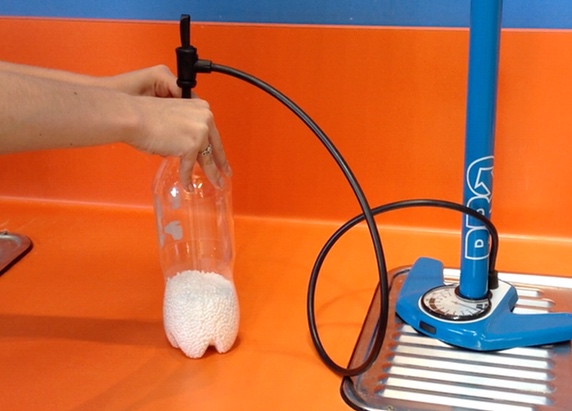
Have one experimenter hold the bottle and connector upright, while the other starts pumping air into the bottle. With each pump, the beads should get noticeably smaller.
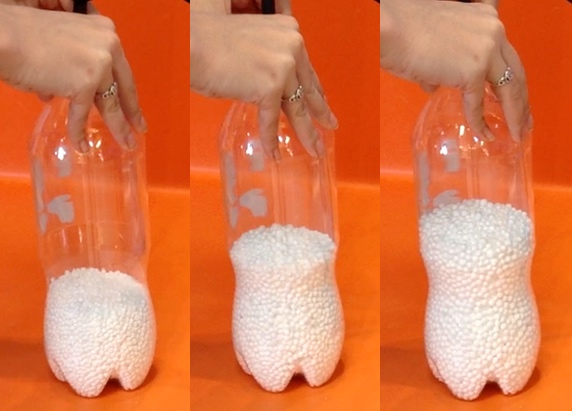
Squeeze the bottle gently to feel how much air is in the bottle. Stop pumping when you can no longer dent or bend the sides of the bottle. Using the mark you made on the side of the bottle, compare the new volume with the starting volume of the beads. What happened?
Holding the bottle firmly, slowly release the connector so air can escape from the bottle. The beads will return to their normal size. Repeat as often as you would like!
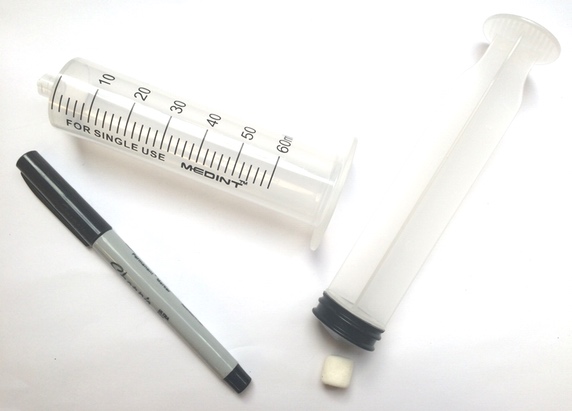
Simulate High and Low Pressure in a Syringe
What happens when you decrease the pressure around an object? Remove the air around a fluffy marshmallow to observe what happens in environments with less pressure (like space).
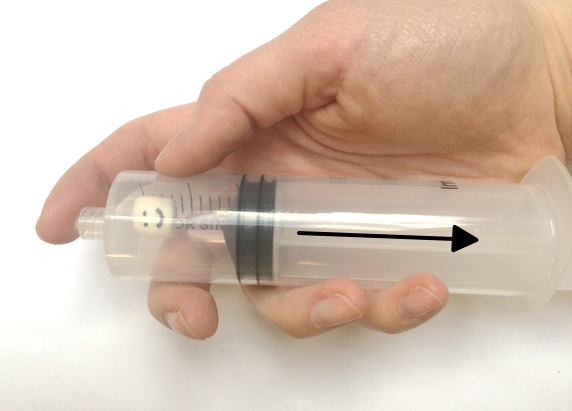
Simulate a low pressure environment:
With the plunger removed, put a marshmallow into the syringe, then push the plunger back into the syringe until it nearly touches the marshmallow. Cover the tip of the syringe with your finger, and then pull the plunger out as far as you can and watch as the marshmallow swells.
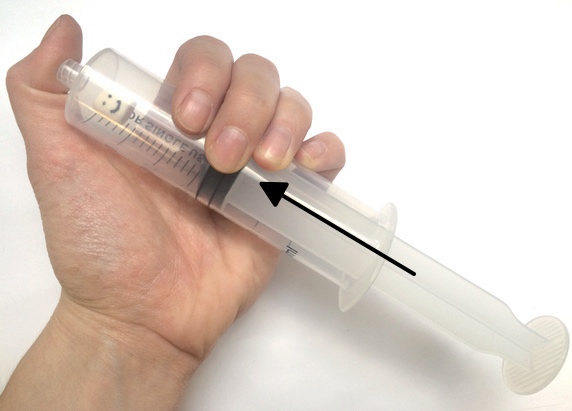
Simulate a high-pressure environment:
Remove the plunger from the syringe completely, leaving the marshmallow inside. Hold your finger firmly over the tip of the syringe and replace the plunger. Without letting any air escape from the tip of the syringe, push down the plunger as hard as you can and watch as the marshmallow shrivels.
Marshmallows, just like EPF cups, contain many small air bubbles, each with their own fixed amount of air pressure. In a low-pressure environment, the pressure inside the tiny air bubbles of a marshmallow (or foam object) is high enough to push outward against the ambient air, causing the bubbles to grow and the marshmallow to expand. But under high ambient pressure, the pressure of the air around the marshmallow is greater than the air trapped inside the marshmallow’s bubbles. As a result, the high-pressure ambient air pushes on the bubbles, compressing the air inside and causing them to shrink. Think of this pressure imbalance as a shoving contest between the air inside the foam and the air outside the foam: whichever has the most pressure will push more strongly and take up more space.
Extension: What happens when you shrink foam cups to different depths?
If pressure underwater increases with depth, then one might predict that if identical cups are sent down to deeper and deeper ocean depths, the cups that travel the deepest will shrink more than the cups that travel to shallower depths. In an original scientific experiment conducted aboard the E/V Nautilus , two members of the Nautilus Corps of Exploration decided to investigate that hypothesis. They created pairs of identical cups, and sent one from each pair to a different depth, leaving the second cup behind as an experimental control. To make it easier to quantitatively measure how much each cup shrank, a 5-centimeter scale was drawn on the side of each cup before it went down.
Each traveling cup descended aboard the ROV Argus to a unique depth—either 650m, 1180m, 1650m, 2200m, or 3300m. The final scale sizes of each cup are reported in the graph below:
Download data here: Raw Foam Cup Data
Use the data to answer the following questions:
- Is there a clear relationship between depth and how much each cup shrank?
- Take a look again at the SEM image of the shrunken foam at the top of this page. Does it provide any hints about the limits to which a foam cup can shrink?
- What do these data tell you about the depth at which the cup cannot shrink any more?
- Why might the amount of shrinkage differ if you were to measure different sides of the same cup? (Hint: Look at the pictures of the cups that were shrunken. How are they different?)
- Math challenge #1: What was the percentage decrease in cup size for each cup pair based on the scale measurements? What is the average percent decrease in the sizes of cups that traveled more than 600 m deep?
- Math challenge #2: Try to re-create this graph so that the x-axis is “pressure” rather than depth. Remember that for every 10 m deeper an ROV travels into the ocean, there is an additional 6.47kg (14.27lbs) of pressure on each square inch of surface.
About This Resource
This resource was created by Rachel Rayner and Ariel Zych inspired by their experience as 2015 Science Communication Fellows aboard the Exploration Vessel (E/V) Nautilus during its July 2015 expedition to the Galápagos Islands. The Science Communication Fellowship Program, an initiative of the Ocean Exploration Trust, brings formal and informal educators together from around the world to support education aboard the E/V Nautilus as members of the Nautilus Corps of Exploration. Fellows are charged with the responsibility of engaging students and the public in the wonders of ocean exploration, sharing discoveries from missions as well as aspects of daily life aboard a working exploration vessel. To learn more about free opportunities for students and teachers to travel aboard the E/V Nautilus as members of the Corps of Exploration visit the Ocean Exploration Trust at www.oceanexplorationtrust.org .
About the Ocean Exploration Trust The Ocean Exploration Trust was founded in 2008 by Dr. Robert Ballard to explore the ocean, seeking out new discoveries in the fields of geology, biology, maritime history, archaeology, physics, and chemistry while pushing the boundaries of STEM education and technological innovation. The Ocean Exploration Trust’s international program is launched from aboard the E/V Nautilus , offering live exploration to participants on shore and the public via live video, audio, and data feeds. Follow us online at www.nautiluslive.org , on Facebook and Instagram @NautilusLive, and on Twitter as @EVNautilus .
Educator's Toolbox
Meet the writers, about ariel zych.
Ariel Zych is Science Friday’s director of audience. She is a former teacher and scientist who spends her free time making food, watching arthropods, and being outside.
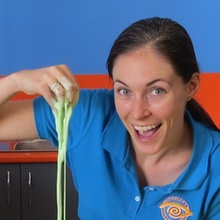
About Rachel Rayner
Rachel Rayner is the Education Coordinator at the Discovery Science and Technology Centre, Bendigo, Victoria (Australia), where she designs educational resources, activities, workshops, shows and events for students and teachers.
Explore More
Looking for life in the deep ocean: an engineering challenge.
How would you look for life in the deepest, darkest parts of the ocean? Get ready to investigate and invent as you search the seas.
ROVs: The Swiss Army Knife Of The Ocean
These versatile machines keep deep sea explorers above water where it's safer, but is operating an ROV really just like operating a big remote control car?
Privacy Overview
Cookie | Duration | Description |
---|---|---|
_abck | 1 year | This cookie is used to detect and defend when a client attempt to replay a cookie.This cookie manages the interaction with online bots and takes the appropriate actions. |
ASP.NET_SessionId | session | Issued by Microsoft's ASP.NET Application, this cookie stores session data during a user's website visit. |
AWSALBCORS | 7 days | This cookie is managed by Amazon Web Services and is used for load balancing. |
bm_sz | 4 hours | This cookie is set by the provider Akamai Bot Manager. This cookie is used to manage the interaction with the online bots. It also helps in fraud preventions |
cookielawinfo-checkbox-advertisement | 1 year | Set by the GDPR Cookie Consent plugin, this cookie is used to record the user consent for the cookies in the "Advertisement" category . |
cookielawinfo-checkbox-analytics | 11 months | This cookie is set by GDPR Cookie Consent plugin. The cookie is used to store the user consent for the cookies in the category "Analytics". |
cookielawinfo-checkbox-functional | 11 months | The cookie is set by GDPR cookie consent to record the user consent for the cookies in the category "Functional". |
cookielawinfo-checkbox-necessary | 11 months | This cookie is set by GDPR Cookie Consent plugin. The cookies is used to store the user consent for the cookies in the category "Necessary". |
cookielawinfo-checkbox-others | 11 months | This cookie is set by GDPR Cookie Consent plugin. The cookie is used to store the user consent for the cookies in the category "Other. |
cookielawinfo-checkbox-performance | 11 months | This cookie is set by GDPR Cookie Consent plugin. The cookie is used to store the user consent for the cookies in the category "Performance". |
csrftoken | past | This cookie is associated with Django web development platform for python. Used to help protect the website against Cross-Site Request Forgery attacks |
JSESSIONID | session | The JSESSIONID cookie is used by New Relic to store a session identifier so that New Relic can monitor session counts for an application. |
nlbi_972453 | session | A load balancing cookie set to ensure requests by a client are sent to the same origin server. |
PHPSESSID | session | This cookie is native to PHP applications. The cookie is used to store and identify a users' unique session ID for the purpose of managing user session on the website. The cookie is a session cookies and is deleted when all the browser windows are closed. |
TiPMix | 1 hour | The TiPMix cookie is set by Azure to determine which web server the users must be directed to. |
viewed_cookie_policy | 11 months | The cookie is set by the GDPR Cookie Consent plugin and is used to store whether or not user has consented to the use of cookies. It does not store any personal data. |
visid_incap_972453 | 1 year | SiteLock sets this cookie to provide cloud-based website security services. |
X-Mapping-fjhppofk | session | This cookie is used for load balancing purposes. The cookie does not store any personally identifiable data. |
x-ms-routing-name | 1 hour | Azure sets this cookie for routing production traffic by specifying the production slot. |
Cookie | Duration | Description |
---|---|---|
__cf_bm | 30 minutes | This cookie, set by Cloudflare, is used to support Cloudflare Bot Management. |
bcookie | 2 years | LinkedIn sets this cookie from LinkedIn share buttons and ad tags to recognize browser ID. |
bscookie | 2 years | LinkedIn sets this cookie to store performed actions on the website. |
lang | session | LinkedIn sets this cookie to remember a user's language setting. |
lidc | 1 day | LinkedIn sets the lidc cookie to facilitate data center selection. |
S | 1 hour | Used by Yahoo to provide ads, content or analytics. |
sp_landing | 1 day | The sp_landing is set by Spotify to implement audio content from Spotify on the website and also registers information on user interaction related to the audio content. |
sp_t | 1 year | The sp_t cookie is set by Spotify to implement audio content from Spotify on the website and also registers information on user interaction related to the audio content. |
UserMatchHistory | 1 month | LinkedIn sets this cookie for LinkedIn Ads ID syncing. |
Cookie | Duration | Description |
---|---|---|
__jid | 30 minutes | Cookie used to remember the user's Disqus login credentials across websites that use Disqus. |
_gat | 1 minute | This cookie is installed by Google Universal Analytics to restrain request rate and thus limit the collection of data on high traffic sites. |
_gat_UA-28243511-22 | 1 minute | A variation of the _gat cookie set by Google Analytics and Google Tag Manager to allow website owners to track visitor behaviour and measure site performance. The pattern element in the name contains the unique identity number of the account or website it relates to. |
AWSALB | 7 days | AWSALB is an application load balancer cookie set by Amazon Web Services to map the session to the target. |
countryCode | session | This cookie is used for storing country code selected from country selector. |
Cookie | Duration | Description |
---|---|---|
_fbp | 3 months | This cookie is set by Facebook to display advertisements when either on Facebook or on a digital platform powered by Facebook advertising, after visiting the website. |
fr | 3 months | Facebook sets this cookie to show relevant advertisements to users by tracking user behaviour across the web, on sites that have Facebook pixel or Facebook social plugin. |
IDE | 1 year 24 days | Google DoubleClick IDE cookies are used to store information about how the user uses the website to present them with relevant ads and according to the user profile. |
NID | 6 months | NID cookie, set by Google, is used for advertising purposes; to limit the number of times the user sees an ad, to mute unwanted ads, and to measure the effectiveness of ads. |
personalization_id | 2 years | Twitter sets this cookie to integrate and share features for social media and also store information about how the user uses the website, for tracking and targeting. |
test_cookie | 15 minutes | The test_cookie is set by doubleclick.net and is used to determine if the user's browser supports cookies. |
vglnk.Agent.p | 1 year | VigLink sets this cookie to track the user behaviour and also limit the ads displayed, in order to ensure relevant advertising. |
vglnk.PartnerRfsh.p | 1 year | VigLink sets this cookie to show users relevant advertisements and also limit the number of adverts that are shown to them. |
VISITOR_INFO1_LIVE | 5 months 27 days | A cookie set by YouTube to measure bandwidth that determines whether the user gets the new or old player interface. |
YSC | session | YSC cookie is set by Youtube and is used to track the views of embedded videos on Youtube pages. |
yt-remote-connected-devices | never | YouTube sets this cookie to store the video preferences of the user using embedded YouTube video. |
yt-remote-device-id | never | YouTube sets this cookie to store the video preferences of the user using embedded YouTube video. |
yt.innertube::nextId | never | This cookie, set by YouTube, registers a unique ID to store data on what videos from YouTube the user has seen. |
yt.innertube::requests | never | This cookie, set by YouTube, registers a unique ID to store data on what videos from YouTube the user has seen. |
Cookie | Duration | Description |
---|---|---|
_dc_gtm_UA-28243511-20 | 1 minute | No description |
abtest-identifier | 1 year | No description |
AnalyticsSyncHistory | 1 month | No description |
ARRAffinityCU | session | No description available. |
ccc | 1 month | No description |
COMPASS | 1 hour | No description |
cookies.js_dtest | session | No description |
debug | never | No description available. |
donation-identifier | 1 year | No description |
f | never | No description available. |
GFE_RTT | 5 minutes | No description available. |
incap_ses_1185_2233503 | session | No description |
incap_ses_1185_823975 | session | No description |
incap_ses_1185_972453 | session | No description |
incap_ses_1319_2233503 | session | No description |
incap_ses_1319_823975 | session | No description |
incap_ses_1319_972453 | session | No description |
incap_ses_1364_2233503 | session | No description |
incap_ses_1364_823975 | session | No description |
incap_ses_1364_972453 | session | No description |
incap_ses_1580_2233503 | session | No description |
incap_ses_1580_823975 | session | No description |
incap_ses_1580_972453 | session | No description |
incap_ses_198_2233503 | session | No description |
incap_ses_198_823975 | session | No description |
incap_ses_198_972453 | session | No description |
incap_ses_340_2233503 | session | No description |
incap_ses_340_823975 | session | No description |
incap_ses_340_972453 | session | No description |
incap_ses_374_2233503 | session | No description |
incap_ses_374_823975 | session | No description |
incap_ses_374_972453 | session | No description |
incap_ses_375_2233503 | session | No description |
incap_ses_375_823975 | session | No description |
incap_ses_375_972453 | session | No description |
incap_ses_455_2233503 | session | No description |
incap_ses_455_823975 | session | No description |
incap_ses_455_972453 | session | No description |
incap_ses_8076_2233503 | session | No description |
incap_ses_8076_823975 | session | No description |
incap_ses_8076_972453 | session | No description |
incap_ses_867_2233503 | session | No description |
incap_ses_867_823975 | session | No description |
incap_ses_867_972453 | session | No description |
incap_ses_9117_2233503 | session | No description |
incap_ses_9117_823975 | session | No description |
incap_ses_9117_972453 | session | No description |
li_gc | 2 years | No description |
loglevel | never | No description available. |
msToken | 10 days | No description |
- Skip to main content
- Keyboard shortcuts for audio player

- LISTEN & FOLLOW
- Apple Podcasts
- Amazon Music
Your support helps make our show possible and unlocks access to our sponsor-free feed.
The High Pressure Experiments That Made D-Day
What happens to the body in the deep sea? You need oxygen to survive, but too much oxygen can be deadly. Also, if you rise to the surface too quickly, nitrogen bubbles can form in your body and kill you. We'll talk with author and scientist Rachel Lance, who has conducted research for the military, using a hyperbaric chamber in which the air and the pressure can be controlled to mimic what divers and submarines are exposed to. Her new book is about the scientists whose dangerous experiments about underwater pressure and injury were critical to the success of D-Day. It's called Chamber Divers . David Bianculli reviews the new series Franklin , starring Michael Douglas as Ben Franklin.
High-Pressure Equipment for Use in the Laboratory, at Sea and at Depth
- First Online: 13 July 2021
Cite this chapter
- Alister Macdonald 2
1015 Accesses
In Chap. 1 , the point was made that the greatest pressure in the oceans, about 110 MPa, is not particularly challenging in engineering terms. It is at the high end of the pressure range used in much hydraulic equipment and industrial machinery. The humble hydraulic car jack and road digger employ pressures in the 35–100 MPa range. However, interesting engineering problems arise in high-pressure biology for lots of reasons. Measurements are required to investigate life processes at high pressure, organisms and cells have to be kept healthy at high pressure, and often they need to be examined with a microscope. Humans wish to explore the depths in submersible craft and see the environment for themselves, human divers need to be housed in hyperbaric chambers for weeks at a time, and in rare circumstances, submariners need to escape from their damaged boat.
This is a preview of subscription content, log in via an institution to check access.
Access this chapter
Subscribe and save.
- Get 10 units per month
- Download Article/Chapter or eBook
- 1 Unit = 1 Article or 1 Chapter
- Cancel anytime
- Available as PDF
- Read on any device
- Instant download
- Own it forever
- Available as EPUB and PDF
- Compact, lightweight edition
- Dispatched in 3 to 5 business days
- Free shipping worldwide - see info
- Durable hardcover edition
Tax calculation will be finalised at checkout
Purchases are for personal use only
Institutional subscriptions
Those in bold can be seen on the web without fee or affiliation. Those marked * are further reading.
Abegg F, Hohnberg H-J, Pape T, Bohrmann G, Freitag J (2008) Development and application of pressure-core-sampling systems for the investigation of gas and gas-hydrate – bearing sediments. Deep-Sea Res I 55:1590–1159
Article Google Scholar
AD 2000-Merkblatt (2019) Technical rules for pressure vessels, Section N4: pressure vessels made of glass. https://www.ad-2000-online.de/en . Accessed Sep 2019 *
Agamat E (1893) Reports on the elasticity and expansibility of liquids at very high pressures. Ann Chim Phys Series 6 29:68–96. *
Google Scholar
Agamat E (1896) Reports on the elasticity and expansibility of liquids at very high pressures. Notice sur les travaux scientifques de M. E. H. Agamat, Paris *
ASME (2015) Boiler and pressure vessel code, Section VIII Rules for construction of pressure vessels, Division 1. American Society of Mechanical Engineers *
ASME (2016a) Safety standard for pressure vessels for human occupancy: ASME PVHO-1-2016. American Society of Mechanical Engineers *
ASME (2016b) Safety standard for pressure vessels for human occupancy: in-service guidelines ASME PVHO-2-2016. American Society of Mechanical Engineers *
Ben-Ari E (2002) Intimate connections: geomicrobiologists explore the interactions between biosphere and geosphere. Bioscience 52:326–331
Bianchi A, Garcin J (1991) In stratified waters the metabolic rate of deep sea bacteria decreases with decompression. Deep-Sea Res I 40:1703–1710
Bianchi A, Garcin J (1993) In stratified waters the metabolic rate of deep sea bacteria decreases with decompression. Deep-Sea Res I 40:1703–1710
Article CAS Google Scholar
Bianchi A, Gardin J, Tholosan O (1999) A high pressure serial sampler to measure microbial activity in the deep sea. Deep-Sea Res I 46:2129–2142
Biggers RE, Chilton JM (1959) Review and bibliography on design and use of windows for optical measurements at elevated temperatures and pressures 1881–1959. Oak Ridge National Laboratory. *
Braeuer A (2015) In situ spectroscopic techniques at high pressure. Elsevier, ISBN 0444634207, 9780444634207. *
Brandt A et al ( 2016 ) Cutting the umbilical: new technological perspectives in benthic deep sea research . J Mar Sci Eng 4 ( 2 ): 36
Bridgman PW (1931) The physics of high pressure. G. Bell and Sons, Ltd. *
Brown DM (1975) Four biological samplers: opening-closing midwater trawl, closing vertical tow net pressure fish trap, free-vehicle drop camera. Deep-Sea Res 22:565–567
Case DH et al (2017) Aerobic and anaerobic methanotropic communities associated with methane hydrates exposed on the seafloor: a high pressure sampling and stable isotope-incubation experiment. Front Microbiol 8:art 2569
Chandra Shekar NV, Sahu PC (2007) High pressure research on materials: 2. Experimental techniques to study the behaviour of materials under high pressure. Resonance 12. https://doi.org/10.1007/s12045-007-0082-6
Chastain RA, Yayanos AA (1991) Ultrastructural changes in an obligately barophilic marine bacterium after decompression. Appl Environ Microbiol 57:1489–1497
Article CAS PubMed PubMed Central Google Scholar
Chervin JC, Syfosse G, Besson JM (1994) Mechanical strength of sapphire windows under pressure. Rev Sci Instrum 65:2719–2725
Cowen JP et al (2003) Fluids from aging ocean crust that support microbial life. Science 299:120–123
Article CAS PubMed Google Scholar
Cowen JP et al (2012) Advanced instrument system for real-time and time-series microbial geochemical sampling of the deep (basaltic) crustal biosphere. Deep Sea Res 61:43–56 *
Cui W (2018) An overview of submersible research and development in China. J Marine Sci Appl 17(4):459–470. https://doi.org/10.1007/s11804-018-00062-6 . *
Cui WC, Hu Y, Guo W, Pan BB, Wang F (2014) A preliminary design of a movable laboratory for hadal trenches. Methods Oceanogr 9:1–16. *
Cui WC, Wang F, Pan BB, Hu Y, Du QH (2016) Issues to be solved in the design, manufacture and maintenance of full ocean depth manned cabin. Adv Eng Res 11:1–29. *
CAS Google Scholar
D’Hondt S, Inagaki F, Ferdelman T, Jørgensen BB, Kato K, Kemp P, Sobecky P, Sogin M, Takai K (2007) Exploring subseafloor life with the integrated ocean drilling program. Scientific Drilling 5:26–37
Damasceno-Oliviera A, Goncalves J, Silva J, Fernandez-Duran B, Coimbra J (2004) A pressurising system for long term study of marine or freshwater organisms enabling the simulation of cyclic vertical migrations. Scientia Marina 68:615–619. *
Das P (2002) Redesign of NEMO-type spherical acrylic submersible for manned operation to 3000 feet (914 m) ocean depth. J Pres Ves Technol 124(1):97. https://doi.org/10.1115/1.1428746 . *
Deguchi S, Tsujii K (2002) Flow cells for in situ optical microscopy in water at temperatures and pressures up to supercritical state. Rev Sci Instrum 73:3938–3941
Delcour AH, Martinac B, Adler J, Kung C (1989) Modified reconstitution method used in patch clamp studies of Escherichia coli ion channels. Biophys J 56:631–636
Deming JW, Somers LK, Straube EL, Swartz DG, Macdonell MT (1988) Isolation of an obligately barophilic bacterium and description of a new genus, Colwellia gen. nov. Syst Appl Microbiol 10:152–160
Dietz AS, Yayanos AA (1978) Silica gel media for isolating and studying bacteria under hydrostatic pressure. Appl Environ Microbiol 36:966–968
Downs JL, Payne RT (1969) A review of electrical feed-through techniques for high pressure gas systems. Rev Sci Instrum 40. *
Drazen JC, Bird LE, Barry JP ( 2005 ) Development of a hyperbaric trap-respirometer for the capture and maintenance of live deep-sea organisms . Limnol Oceanogr Methods 3 ( 2005 ): 488 – 498
Evans S, Birch J, Brier J, Jakuba M, Salto M, Robidart J (2019) Ocean robots uncover microbial secrets. Microbiol Society Oceans 5 Feb
Faes, W., Lecompte, S., Van Bael, J., Salenbien, R., Bäßler, R., Bellemans, I., Cools, P., De Geyter, N., Morent, R., Verbeken, K., De Paepe, M. (2019, November) Corrosion behaviour of different steel types in artificial geothermal fluids Geothermics Volume 82, 182–189. *
Frey B et al ( 2006 ) Microscopy under pressure – an optical chamber system for fluorescence microscopic analysis of living cells under high hydrostatic pressure . Microsc Res Tech 69 : 65 – 72
Article PubMed Google Scholar
Friedrich O, Wegner FV, Hartmann M, Frey B, Sommer K, Ludwig H, Fink RHA (2006) In situ high pressure confocal Ca ++ fluorescence microscopy in skeletal muscles: a new method to study pressure limits in mammalian cells. Undersea Hyperbaric Med 33:181–195
Fryer DM, Harvey JF (2012) High pressure vessels. Springer Science & Business Media
Garel M et al ( 2019 ) Pressure-retaining sampler and high pressure systems to study deep sea microbes under in situ conditions . Front Microbiol 10 : art 453
Gibbon HA (1968) Pressure liquid sampling system and apparatus. U.S. Patent No 3,379, 065, 1968 *
Gilchrist I (1972) Equipment for the recovery and study of deep sea animals. PhD thesis, The Queens University of Belfast, UK
Gilchrist I, Macdonald AG (1983) Techniques for experiments with deep sea animals. In: Macdonald AG, Priede IG (eds) Experimental biology at sea. Academic Press, London
Giovanelli D, Lawrence NS, Compton RG ( 2004 ) Electrochemistry at high pressures: a review . Electroanalysis 16 : 789 – 810
Grasset O (2001) Calibration of the R ruby fluorescence lines in the pressure range (0–1 GPa) and the temperature range (250–300 K). High Pressure Res 21:139–157
Gundersen KR, Mountain CW (1972) A pressure and temperature preserving sampling, transfer, and incubation system for deep sea microbiological and chemical research. In: Brauer RW (ed) Barobiology and the experimental biology of the deep sea. University of Northe Carolina, USA. *
Guorui L et al (2021) Self-powered soft robot in the Mariana Trench. Nature 591:66–71 *
Haberl B, Molaison JJ, Neuefeind JC, Luke L, Daemen LL, Boehler R ( 2019 ) Modified Bridgman anvils for high pressure synthesis and neutron scattering . High Pressure Res 39 : 426 – 437
Halle D, Yedgar S (1988) Mild pressure induces resistance of erythrocytes to hemolysis by snake venom phospholipase A2. Biophys J 54:393–396. *
Harper AA, Macdonald AG, Wardle CS, Pennec J-P (1987) The pressure tolerance of deep sea fish axons; Results of Challenger Cruise 6/b85. Comp
Hauge JG (1971) Pressure induced dissociation of ribosomes during ultracentrifugation. FEBS Lett 17:168–172
Haver T, Raber EC, Urayama P (2006) An application of spatial deconvolution to a capillary high pressure chamber for fluorescence microscopy microscope imaging. J Microsc 230:363–371
Heden CG (1964) Effects of hydrostatic pressure on microbial systems. Bacteriol Rev 28:14–29. *
Heinemann SH, Stuhmer W, Conti F ( 1987 ) Si9ngle acetylcholine receptor channel currents recorded at high hydrostatic pressures . Proc Natl Acad Sci U S A 84 : 3229 – 3233
Hemley R (2010) Percy W. Bridgman’s second century. High Pressure Res 30:581–689
Hodge A, Fujan RS, Carlson KL, Burgess RG, Harris WH, Mann RW ( 1986 ) Contact pressures in the human hip joint measured in vivo . Proc Natl Acad Sci U S A 83 : 2879 – 2883
Holtgrewe N, Greenberg E, Prescher C, Prakapenka VB, Goncharov AF (2019) Advanced integrated optical spectroscopy system for diamond anvil cell studies at GSECARS. High Pressure Res 39(3):457–470. *
Huey D, Storms M (1985) The ocean drilling program IV: deep water coring technology-past, present, and future. Published in: OCEANS ’85 – Ocean Engineering and the Environment, 12–14 Nov
Humphris S, Soule A (2019) Vehicles for deep sea exploration. Encyclopedia of Ocean Sciences, Academic Press
Inoue S, Fuseler J, Salmon ED, Ellis GW (1975) Functional organization of mitotic microtubules. Bioophys J 15:725–744
Ishii A, Nishiyama M, Yanagida T (2004) Mechano-chemical coupling of molecular motors revealed by single molecule measurements. Curr Protein Pept Sci 5:81–87
Jackson K, Witte U, Chalmers S, Anders E, Parkes J ( 2017 ) A system for retrieval and incubation of benthic sediment cores at in situ ambient pressure and under controlled or manipulated environmental conditions . J Atmos Oceanic Tech 34 : 983 – 1000
Jannasch HW, Wirsen CO ( 1977 ) Retrieval of concentrated and undecompressed microbial populations from the deep sea . Appl Environ Microbiol 33 : 642 – 646
Jannasch HW, Wirsen CO, Winget CL (1973) Bacteriological pressure-retaining deep-sea sampler and culture vessel. Deep-Sea Res 20:661–662
Jannasch HW, Wirsen CO, Taylor CD ( 1976 ) Undecompressed microbial populations from the deep sea . Appl Environ Microbiol 32 : 360 – 367
Jannasch HW, Wirsen CO, Taylor CD (1982) Deep sea bacteria: isolation in the absence of decompression. Science 216:1315–1316
Jin Y, Konno Y, Nagao J (2014) Pressurised subsampling system for pressurised gas-hydrate-bearing sediment: microscale imaging using X-ray computed tomography. Rev Sci Instrum 85:094502
Article PubMed CAS Google Scholar
Jose A, Caro A, Wand J ( 2018 ) Practical aspects of high pressure NMR spectroscopy and its applications in protein biophysics and structural biology . Methods 148 : 67 – 80
Josephs R, Harrington WF (1966) Studies on the formation and physical chemical properties of synthetic myosin filaments. Biochemistry 5:3474–3487. *
Josephs R, Harrington WF (1967) Sedimentary studies on interacting systems: pressure dependence of the polymerization of myosin. Fed Proc 26:abstract 2630. *
Kato C, Nogi Y, Arakawa S (2008) Isolation, cultivation and diversity of deep sea piezophiles. Chapter 12. In: Michiels C, Bartlett DH, Aertsen A (eds) High pressure microbiology. ASM Press, Washington, DC
Kegeles G, Rhodes L, Bethune JL (1967) Sedimentation behaviour of chemically reacting systems. Proc Natl Acad Sci U S A 58:45–51. *
Kemper B, Kemper K (2019) NEMO joint design in the ASME PVHO Code. Presented at 16th Annual Manned Underwater Vehicles Symposium (Marine Technology Society)
Kitching JA ( 1954 ) The effects of high pressure on a suctorian . J Exp Biol 31 : 56
Koyama S (2007) Cell biology of deep sae multicellular organisms. Cytotechnology 55:125–133
Article PubMed PubMed Central Google Scholar
Koyama S, Miwa T, Sato T, Aizawa M ( 2001 ) Optical chamber system designed for microscopic observation of living cells under extremely high hydrostatic pressure . Extremophiles 5 : 409 – 415
Koyama S et al (2002) Pressure-stat aquarium system designed for capturing and maintaining deep sea organisms. Deep-Sea Res I 49:2095–2102
Koyama S et al ( 2005 ) Survival of deep sea shrimp (Alvinocaris sp.) during decompression and larval hatching at atmospheric pressure . Marine Biotechnol 7 : 272 – 278
Kusube M et al (2017) Colwellia marinimaniae sp. nov., a hyperpiezophilic species isolated from an amphipod within the Challenger Deep, Mariana Trench. Int J Syst Evol Microbiol 67:824–831
Kyo M, Tuji T, Usui H, Itoh T (1991) Collection, isolation and cultivation system for deep-sea microbes study: concept and design. Oceans 1:419–423
Landau JV, Thibodeau L (1962) The micromorphology of Amoeba proteus during pressure-induced changes in the sol-gel cycle. Exp Cell Res 27:591–594
Lemmon EW, Hubner ML, McLinden MO (2010) NIST standard reference database 23: reference fluid thermodynamic and transport properties-REFPROP.9.0
Lerch MT, Yang Z, Brocks EK, Hubbell WL ( 2014 ) Mapping protein conformational heterogeneity under pressure with site directed spin labelling and double electron-electron resonance . Proc Natl Acad Sci : E1201 –E 1210
Li L et al (2016) Pressure retaining method based onphase change forcoring of gas hydrate-bearing sediments in offshore drilling. Appl Thermal Eng 107:633–641
Li Y, Peng J, Huang C, Wang M (2019) Experimental study on a sampling technique based on a freeze-sediments valve for deep sea microorganisms. Appl Ocean Res 82:470–477. *
Lipp MJ, Evans WJ, Yoo CS ( 2005 ) Hybrid Bridgman anvil design: an optical window for in situ spectroscopy in large volume presses . High Pressure Res 25 : 205 – 210
Liu PF, Wang SB, Li XKJ (2018) Finite element analysis of failure behaviors of the inspection window of the deep-sea submersible. Fail Anal Preven 18:1198
Macdonald AG ( 1997 ) Effects of high hydrostatic pressure on the BK channel in bovine chromaffin cells . Biophys J 73 : 1866 – 1873
Macdonald AG, Gilchrist I (1969) Recovery of deep sea water at constant pressure. Nature 222:71–72
Macdonald AG, Gilchrist I (1972) An apparatus for the recovery and study of deep sea plankton at constant temperature and pressure. In: Brauer RW (ed) Baro-biology and the experimental biology of the deep sea. University of North Carolina Press, pp 394–407
Macdonald AG, Gilchrist I (1978) Further studies on the pressure tolerance of deep sea crustacea with observations using a new high pressure trap. Mar Biol 45:9–21
Macdonald AG, Gilchrist I (1982a) The pressure tolerance of deep sea Amphipods collected at their ambient high pressure. Comp Biochem Physiol 71A:348–352. *
Macdonald AG, Gilchrist I (1982b) The pressure tolerance of Amphipods collected at their ambient high pressure. Comp Biochem Physiol 71A:349–352. *
Macdonald AG, Martinac B ( 1999 ) Effect of high hydrostatic pressure on the porin Omp C from Escherichia coli . FEMS Microbiol Lett 173 : 329 – 334
Macdonald AG, Vjotosh AN (1999) Patch-clamp recording of BK (Ca) channels in hyperbaric oxygen. J Physiol 51P:111P
Macdonald AG, Gilchrist I, Wann KT, Wilcock SE (1979) The tolerance of Animals to pressure. In: Gilles R (ed) Animals and environmental fitness, vol 1. Pergamon Press. *
Macdonald AG, Ramsey RL, Shelton CJ, Usherwood PNR (1989) An apparatus for single channel patch recording at high pressure. J Physiol 409:2P
Malahoff A, Gregory T, Bossuyt A, Donachie S, Alam M (2002) A seamless system for the collection and cultivation of extremophiles from the deep ocean. IEEE J Ocean Eng 27:862–869
Maltas J, Long Z, Huff A, Maloney R, Ryan J, Urayama P (2014) A micro-perfusion system for use during real-time physiological studies under high pressure. Rev Sci Instrum 85:106106
Marsland D ( 1958 ) Cells at high pressure . Sci Am 199 : 36 – 43
Marsland D (1970) Pressure–temperature studies on the mechanisms of cell division. In: Zimmerman A (ed) High pressure effects on cellular processes. Academic Press, pp 259–312. *
Masui N, Kao C (1999) New method of screening for pressure sensitive mutants at high hydrostatic pressure. Biosci Biotechnol Biochem 63:235–237
Mavor JW (1965) Observation windows of the deep submersible, ALVIN (AD0629592). Technical Report 65–62. Woods Hole Oceanographic Institution, Woods Hole (MA). *
Book Google Scholar
Mayer A (2012) Life in the deep biosphere. Bioscience 62:453–457
McCoy J, Hubbell WL ( 2011 ) High pressure EPR reveals conformational equilibria and volumetric properties of spin-labeled proteins . Proc Natl Acad Sci 108 : 1331 – 1336
McNamee MJ, Wawersik WR, Shields ME, Holcomb DJ (1991) A compact coaxial electrical feedthrough for use up to 400 MPa. Rev Sci Instrum 62:1662. *
McNichol J et al (2016) Assessing microbial processes in deep-sea hydrothermal systems via incubations at in situ temperature and pressure. Deep-Sea Res I 115:221–231
McNichol J et al (2018) Primary productivity below the sea floor at deep sea hot springs. Proc Natl Acad Sci U S A 115:1–6. *
Mertens-Strljthagen J, De Schryver C, Wattiaux-De Conninck S, Wattiaux R (1985) The effect of pressure on fetal and neonatal liver mitochondrial membranes. Arch Biochem Biophys 236:825–831. *
Mickel TJ, Childress JJ (1982) Effects of pressure and pressure acclimation on activity and oxygen consumption in the bathypelagic mysid Gnathophausia ingens. Deep-Sea Res 29:1293–1301. *
Miller JB, Aidley JS, Kitching JA ( 1975 ) Effects of helium and other inert gases on Echinosphaerium nucleofilum (Protozoa, Heliozoa) . J Exp Biol 63 : 467 – 481
Mizuno S (2005) A novel method for assessing effects of hydrostatic fluid pressure on intracellular calcium: a study with bovine articular chondrocytes. Am J Physiol Cell Physiol 288:C329–C337
Morrell KC, Hodge WA, Krebs DE, Mann RW ( 2005 ) Corroboration of in vivo cartilage pressure with implications for synovial joint tribology and oateoarthritis causation . Proc Natl Acad Sci U S A 102 : 14819 – 14824
Murayama M (1987) Compression inhibits aggregation of human platelets under high hydraulic pressure. Thromb Res 45:729–738
Nakayama A, Yano Y, Yoshida K (1994) New method for isolating barophiles from intestinal contents of deep sea fishes retrieved from the abyssal zone. Appl Environ Microbiol 60:4210–4212
Nishiyama M (2015) High pressure microscopy for studying molecular motors. Chapter 27. In: Akasaka K, Matsuki H (eds) High pressure bioscience. Springer Science+Business Media Dordrecht
Nishiyama M ( 2017 ) High pressure microscopy for tracking dynamic properties of molecular machines . Biophys Chem 231 : 71 – 78
Nishiyama M, Kojima S (2012) Bacterial motility measured by a miniature chamber for high pressure microscopy. Int J Mol Sci 13:9225–9239. *
Nishiyama M, Sowa Y (2012) Microscopic analysis of bacterial motility at high pressure. Biophys J 102:1872–1880. *
Nishiyama M, Kimura Y, Nishiyama Y, Terazima M (2009) Pressure-induced changes in the structure and function of the kinesin-microtubule complex. Biophys J 96:1142. *
Nishiyama M, Kato C, Abe F (2012) Intracellular pressure measurement by using pressure sensitive Yellow fluorescent protein. Biophys J 102:419a. *
Noji H, Yasuda R, Yoshida M, Kinosita K ( 1997 ) Direct observation of the rotation of F1-ATPase . Nature 386 : 299 – 302
Oger PM, Daniel I, Piccard A (2006) Development of a low pressure diamond anvil cell and analytical tools to monitor microbial activities in situ under controlled P and T. Biochim Biophys Acta 1764:434–442
Orcutt B, Wheat CG, Edwards KJ ( 2010 ) Subseafloor ocean crust microbial observatories: development of FLOCS (Flow through Osmo Colonization System) and evaluation of borehole construction materials . Geomicrobiol J 27 : 143 – 157
Pagliaro L, Reitz F, Wang J (1995) An optical pressure chamber designed for high numerical aperture studies on adherent living cells. Undersea Hyperbaric Med 22:171–181
Paladini AA, Weber G (1981) Absolute measurements of fluorescence polarisation at high pressures. Rev Sci Instrum 52:419–427
Parkes RJ et al ( 2009 ) Culturable prokaryotic diversity of deep, gas hydrate sediments: first use of a continuous high-pressure, anaerobic, enrichment and isolation system for subseafloor sediments (DeepIsoBUG) . Environ Microbiol 11 : 3140 – 3153
CAS PubMed PubMed Central Google Scholar
Peoples LM et al (2019) A full ocean depth rated modular lander and pressure retaining sampler capable of collecting hadal-endemic microbes under in situ conditions. Deep-Sea Res 143:50–57. *
Peterson RW, Wand AJ (2005) Self contained high pressure cell, apparatus and procedure for the preparation of encapsulated proteins dissolved in low viscosity fluids for NMR spectroscopy. Rev Sci Instrum 76:094101
Phillips H, Wells LE, Johnson RV, Elliott S, Deming JW (2003) LAREDO: a new instrument for sampling and in situ incubation of deep sea hydrothermal vent fluids. Deep-Sea Res I 50:1375–1387
Phleger CF, McConnaughey RR, Grill P (1979) Hyperbaric fish trap operation and deployment in the deep sea. Deep-Sea Res 26A:1405–1409
Piccard J, Dietz RS (1957) Oceanographic observations by the Bathyscaph Trieste (1953–1956). Deep-Sea Res 4:221–229. *
Pickles DM, Ogston D, Macdonald AG ( 1990 ) Effects of hydrostatic pressure and inert gases on platelet aggregation in vitro . J Appl Physiol 69 : 2239 – 2247
Poulter TC (1930) A glass window mounting for withstanding pressures of 30,000 atmospheres. Phys Rev 35:297. *
Poulter TC (1932) Apparatus for optical studies at high pressures. Phys Rev 40:860–871. *
Poulter TC, Benz CA (1932) The lens effect of pressure windows. Phys Rev 40:872–876. *
Poulter TC, Buckley F (1932) Diamond windows for withstanding very high pressures. Phys Rev 41:364–365. *
Poulter TC, Wilson RO (1930) Some recent developments in high pressure windows. Proc Iowa Acad Sci 37:259–302. *
Poulter TC, Wilson RO (1932) The permeability of glass and fused quartz to ether, alcohol and water at high pressures. Phys Rev 40:877–880. *
Pradillon F, Shillito B, Chervin J-C, Hamel G, Gaill F (2004) Pressure vessels for in vivo studies of deep sea fauna. High Pressure Res 24:237–246
Qinghai D, Yong H, Weicheng C (2017) Safety assessment of the acrylic conical frustum viewport structure for a deep-sea manned submersible. Ships Offshore Struct 12(sup1):S221–S229. *
Quetin LB, Childress JJ ( 1980 ) Observations on the swimming activity of two bathypelagic mysid species maintained at high hydrostatic pressure . Deep-Sea Res 27 : 383 – 391
Raber EC, Dudley JA, Salerno M, Urayama P (2006) Capillary based, high pressure chamber for fluorescence microscopy imaging. Rev Sci Instrum 77:096106
Rabinowitz S, Ward IM, Parry JSC (1970) The effect of hydrostatic pressure on the shear yield of polymers. J Mater Sci 5:29–39. *
Ranatunga KW, Fortune NS, Geeves MA ( 1990 ) Hydrostatic compression in glycinerated rabbit muscle fibers . Biophys J 58 : 1401 – 1410
Ravaux J et al (2013) Thermal limit for metazoan life in question: in vivo heat tolerance of the Pompeii worm. Plos One 8(e):64074
Ravaux J, Léger N, Hamel G, Shillito B (2019) Assessing a species thermal tolerance through a multiparameter approach: the case study of the deep-sea hydrothermal vent shrimp Rimicaris exoculate. Cell Stress Chaperones 24:647–659
Rebhun LI, Mellon M, Jemiolo D, Nath J, Ivy N (1974) Regulation of size and biretringence of the in vivo mitotic apparatus. J Supramol Struct 2:466–485
Regnard P (1884a) Effekts des hautes pressions sur les animaux marins. Compt Rend Soc Biol 36:394–395. *
Regnard P (1884b) Recherches experimentales sur l’influence de tres haute pressions sur les organisimes vivantes. Comptes Rendues Acad Sci Paris 98:735–747. *
Regnard P (1884c) Note sur les conditions de la vie dans les profondeurs de la mer Comptes. Rend Soc Biol Paris 36:164–168. *
Regnard P (1891) Recherches Experimentales sur les conditions physiques de la Vie dans les Eaux. Masson, Paris. *
Robinson NJ, Thatje S, Osseforth C (2009) Heartbeat sensors under pressure: a new method for assessing hyperbaric physiology. High Pressure Res 29:422–430
Roche J, Royer CA, Roumestand C (2017) Monitoring protein folding through high pressure NMR spectroscopy. Prog Nucl Magn Reson Spectrosc 102–103:15–31. *
Salmon ED (1975a) Pressure induced depolymerization of spindle microtubules I. J Cell Biol 65:603–614. *
Salmon ED (1975b) Pressure induced depolymerization of spindle microtubules II. J Cell Biol 66:114–127. *
Salmon ED (1975c) Spindle microtubules: thermodynamics of in vivo assembly and role in chromosome movement. Ann N Y Acad Sci 253:383–406. *
Salmon ED (1975d) Pressure induced depolymerization of brain microtubules in vitro. Science 189:884–886. *
Salmon ED, Ellis GW ( 1975 ) A new miniature hydrostatic pressure chamber for microscopy . J Cell Biol 65 : 587 – 602
Salmon ED, Goode D, Maugel TK, Bonar DB (1976) Pressure induced depolymerization of spindle microtubules III. J Cell Biol 69:443–454. *
Sauer P, Glombitza C, Kallmeyer J (2012) A system for incubations at high gas partial pressure. Front Microbiol 3:art 25. *
Schmalwasser H, Neef A, Elliott AA, Heinemann SF (1998) Two electrode voltage clamp of Xenopus oocytes under high hydrostatic pressure. J Neurosci Methods 81:1–7
Schneidereit D, Vass H, Reischi B, Allen RJ, Friedrich O ( 2016 ) Calcium sensitive fluorescent dyes Fluo 4 and Fura Red under pressure: behaviour of fluorescence and buffer properties under hydrostatic pressures up to 200 MPa . Plos One 11 : 0164509
Schultheiss PJ, Francis TJG, Holland M, Jackson PD et al (2006) Pressure coring, logging and subsampling with the HYACINTH system. Geol Soc Lond Spec Publ 267:151–163
Seewald JS, Doherty KW, Hammar TR, Liberatore SP (2001) A new gas tight isobaric sampler for hydrothermal fluids. Deep-Sea Res I 49:189–196
Shelton DP (1992) Lens induced by stress in optical windows for high-pressure cells. Rev Sci Instrum 63:3978. https://doi.org/10.1063/1.1143248 . *
Sherman WF, Stadmuller AA (1988) Experimental techniques in high pressure research, 1st edn. Wiley. ISBN-10: 0471103136 ISBN-13: 978-0471103134
Shillito B et al (2001) Temperature resistance of Hesiolyra bergi, a polychaetous annelid living on deep sea vent smoker walls. Mar Ecol Prog Ser 216:141–149
Shillito B, Hamel G, Duchi C, Cottin D, Sarrazin J, Sarradin P-M, Ravaux J, Galli F (2008) Live capture of megafauna from 2300 m depth, using a newly designed pressurized recovery device. Deep-Sea Res I 55:881–889
Shillito B, Gaill F, Ravaux J (2014) The IPOCAMP pressure incubator for deep-sea fauna. J Mar Sci Technol 22:97–102
Skelton WJ, Crossland B (1980) High pressure safety: a review of high pressure equipment development at the Queen’s University of Belfast. Int J Pres Ves Piping 8(1980):377–404
Smiley JE, Drawbridge MA (2007) Techniques for live capture of deepwater fishes with special emphasis on the design and application of a low-cost hyperbaric chamber. J Fish Biol 70:867–878. *
Snoey MR, Katona MG (1970) Structural design of conical acrylic viewports. Technical Report No.: R-686. Naval Civil Engineering Laboratory, Port Hueneme (CA)
Stachiw JD (1966) Critical pressure of conical acrylic windows under short term hydrostatic loading A.S.M.E. Paper 66-WA/UNT.2
Stachiw JD (1967) Windows for external or internal hydrostatic pressure vessels. Part 2: Flat acrylic windows. Technical Report R527 U.S. Naval Civil Engineering Laboratory *
Stachiw JD (1970) Conical; acrylic windows under long term hydrostatic pressure of 20,000 lbf/in2 Trans A.S.M.E. J Eng Ind 92:237–256
Stachiw JD, Gray KO (1967) Windows for external or internal hydrostatic pressure vessels. Part 1: Conical acrylic windows. Technical Report R512. U.S. Naval Civil Engineering Laboratory *
Stokes MD, Somero GN (1999) An optical oxygen sensor and reaction vessel for high pressure applications. Limnol Oceanogr 44:189–195
Sun W et al (2018) Adiabatic compression heating of selected organic solvents under high pressure processing. High Pressure Res 38:325–336
Szafrinski KM, Piquet B, Shillito B, Lallier F, Duperron S (2015) Relative abundance of methane- and sulfur-oxidizing symbionts in gills of the deep sea hydrothermal vent mussel Bathymodiolus azoricus under pressure. Deep-Sea Res I 101:7–13
Tabor PS et al (1981) A pressure-retaining deep ocean sampler and transfer system for measurement of microbial activity in the deep sea. Microb Ecol 7:51–65
Taylor CD et al (2006) Autonomous Microbial Sampler (AMS), a device for the uncontaminated collection of multiple microbial samples from submarine hydrothermal vents and other aquatic environments. Deep-Sea Res I 53:894–916. *
Tekmen M, Muller JD (2004) High pressure cell for fluorescence fluctuation spectroscopy. Rev Sci Instrum 75:5143–5148
Testemale D, Prat A, Lahera E, Hazemann J-L (2016) Novel high-pressure windows made of glass-like carbon for X-ray analysis. Rev Sci Instrum 87:075115
Tian CL, Hu Y, Liu DQ, Cui WC (2010) Creep analysis on deep-sea structure’s viewport windows. J Ship Mech 14(5):526–532. *
Turner A (2016) The evolution of thicker & more complex acrylic windows for manned submersibles. In: Proceedings of the 13th annual manned underwater vehicles symposium (Marine Technology Society). New Orleans, LA. http://www.underwaterintervention.com . Accessed Sep 2019 *
Undersea and Hyperbaric Medical Society (2004) Hyperbaric facility design guidelines version 1.0 July 2004. UHMS Associates. https://www.uhms.org/images/Safety-Articles/aia_facility_design_1_2.pdf . Accessed Sep 2019 *
Undersea and Hyperbaric Medical Society (2012) Safe design and operation of hyperbaric chambers. Reviewed by the UHMS Hyperbaric Oxygen Safety Committee. https://www.uhms.org/images/Safety-Articles/safe_design_and_operation_of.pdf . Accessed Sep 2019 *
Undersea and Hyperbaric Medical Society (2019) Safety references. https://www.uhms.org/publications/safety-documents/safety-references.html . Accessed Sep 2019 *
United Kingdom Department for Business, Energy and Industrial Strategy (2016) Pressure equipment (safety) regulations 2016. https://assets.publishing.service.gov.uk/government/uploads/system/uploads/attachment_data/file/640795/nlf-pressure-equipment-regulations-2016-guidance.pdf . Accessed Sep 2019
Usui K, Hiraki T, Kwamoto J, Kurihara T, Nogi Y (2012) Eicosapentaenoic id plays a role in stabilising dynamic membrane structure in the seep-sea piezophile Shewanella violacea: a study employing high-pressure time-resolved fluorescence anisotropy measurement. Biochim Biophys Acta 1818:574–583. *
Vass H, Lucas-Black SL, Herzig EM, Ward FB, Clegg PS, Allen RJ (2010) A multipurpose modular system for high-resolution microscopy at high hydrostatic pressure. Rev Sci Instrum 81:053710. *
Vass H et al (2013) Single molecule imaging at high hydrostatic pressure. Appl Phys Lett 102:154103
Wahl W (1913a) Physio-chemical determination at high pressure by optical methods, I. Apparatus for optical determinations at high pressures. Trans Roy Soc A 212:117–148. *
Wahl W (1913b) Optische Untersuchung verfestigter Gase. II. Zeitschrift für Physikalische Chemie 84U(1):112–122. *
Wang Y et al (2016) Genomic characteriuzation of symbiotic mycoplasmas from the stomach of deep sea isopod Bathynomus sp. Environ Microbiol 18:2646–2659
Wang F, Wang W, Zhang Y, Du Q, Jiang Z, Cui W (2019) Effect of temperature and nonlinearity of P.M.M.A. material in the design of observation windows for a full ocean depth manned submersible. Mar Technol Soc J 53:27–36
Wann KT, Macdonald AG, Harper AA, Wilcock SE (1979) Electrophysiological measurements at high hydrostatic pressure: methods for intracellular recording from isolated ganglia and for extracellular recording in vivo. Comp Biochem Physiol 64A:141–147
Wilcock SE (1979) The effects of high hydrostatic pressure on a marine crustacean, on the crustacean abdominal nerve cord and another nerve bundle. PhD thesis, Aberdeen University, UK *
Wilcock SE, Wann KT, Macdonald AG (1978) The motor activity of Crangon crangon subjected to high hydrostatic pressure. Mar Biol 45:1–7. *
Wilson RR, Smith KL (1985) Live capture, maintenance and partial decompression of a deep sea grenadier fish (Coryphaenoides acrolepis) in a hyperbaric trap-aquarium. Deep-Sea Res 32:1571–1582. *
Winter R (2002) Synchrotron X-ray and neutron small-angle scattering of lyotropic lipid mesophases, model biomembranes and proteins in solution at high pressure. Biochim Biophys Acta 1595:160–184
Wu W, Chong PL-G, Huang CH (1985) Pressure effect on the rate of crystalline phase formation of L-a-dipalmitoyl phosphatidylcholine in multilamellar dispersions. Biophys J 27:327–342. *
Wu S-J, Wang S, Yang C-J (2018) An isobaric sample transfer apparatus for deep-sea pressurized fluid sample. Rev Sci Instrum 89:086110
Yayanos AA (1977) Simply actuated closure for a pressure vessel: design for use to trap deep sea animals. Rev Sci Instrum 48:786–789
Yayanos AA (1978) Recovery and maintenance of live amphipods at a pressure of 580 bars from an ocean depth of 5700 meters. Science 200:1056. *
Yayanos AA (1995) Microbiology to 10,500 meters in the deep sea. Annu Rev Microbiol 49:777–805. *
Yayanos AA, DeLong EF (1987) Deep sea bacterial fitness to environmental temperatures and pressures. In: Jannasch HW, Marquis RE, Zimmerman AM (eds) Current perspectives in high pressure biology. Academic Press, Orlando, FL. *
Yayanos AA, Dietz AS (1983) Death of a hadal deep sea bacterium after decompression. Science 220:497–498
Yayanos AA, Dietz AS, Von Boxtell R (1981) Obligately barophilic bacterium from the Mariana trench. Proc Natl Acad Sci U S A 78:5212–5215
Yoder P, Vukobratovich D (eds) (2017) Opto-mechanical systems design, Volume 1: Design and analysis of opto-mechanical assemblies. CRC Press, ISBN 1482257718, 9781482257717. *
Zhang J, Zuo X, Wang W, Tang W (2014) Overviews of investigation on submersible pressure hulls. Adv Nat Sci 7(4):1–8. https://doi.org/10.3968/6129 . ISSN 1715-7870 *
Zhang Z, Wu Y, Zhang X-H (2018) Cultivation of microbes from the deep sea environments. Deep-Sea Res II 155:34–43
Zhu Y, Liang W, Zhao X, Wang X, Xia J (2019, Sep) Strength and stability of spherical pressure hulls with different viewport structures. Int J Pres Ves Piping 176. https://doi.org/10.1016/j.ijpvp.2019.103951 . *
Download references
Author information
Authors and affiliations.
Emeritus Reader in Physiology, University of Aberdeen, Kings College, Aberdeen, UK
Alister Macdonald
You can also search for this author in PubMed Google Scholar
Rights and permissions
Reprints and permissions
Copyright information
© 2021 Springer Nature Switzerland AG
About this chapter
Macdonald, A. (2021). High-Pressure Equipment for Use in the Laboratory, at Sea and at Depth. In: Life at High Pressure. Springer, Cham. https://doi.org/10.1007/978-3-030-67587-5_13
Download citation
DOI : https://doi.org/10.1007/978-3-030-67587-5_13
Published : 13 July 2021
Publisher Name : Springer, Cham
Print ISBN : 978-3-030-67586-8
Online ISBN : 978-3-030-67587-5
eBook Packages : Biomedical and Life Sciences Biomedical and Life Sciences (R0)
Share this chapter
Anyone you share the following link with will be able to read this content:
Sorry, a shareable link is not currently available for this article.
Provided by the Springer Nature SharedIt content-sharing initiative
- Publish with us
Policies and ethics
- Find a journal
- Track your research
Thank you for visiting nature.com. You are using a browser version with limited support for CSS. To obtain the best experience, we recommend you use a more up to date browser (or turn off compatibility mode in Internet Explorer). In the meantime, to ensure continued support, we are displaying the site without styles and JavaScript.
- View all journals
- Explore content
- About the journal
- Publish with us
- Sign up for alerts
- Open access
- Published: 07 November 2022
Design and experimental research of a novel deep-sea self-sustaining profiling float for observing the northeast off the Luzon Island
- Qiang Wang 1 , 2 , 4 ,
- Zurong Qiu 2 ,
- Shaobo Yang 3 , 4 ,
- Hongyu Li 3 , 4 &
- Xingfei Li 2 , 4
Scientific Reports volume 12 , Article number: 18885 ( 2022 ) Cite this article
1210 Accesses
1 Citations
2 Altmetric
Metrics details
- Mechanical engineering
- Physical oceanography
To understand the physical ocean laws of ocean circulation in the deep ocean below 2000 m, a profiling float named FUXING is presented to meet the deep-ocean observation requirements at a depth of 4000 m. First, to meet the low energy consumption and buoyancy regulation stability of the profiling float, the low–power buoyancy adjustment process of FUXING is effectively solved by introducing the external seawater pressure as the driving force. Then, to reduce the energy consumption of the single profile for the profiling float, the optimization of the oil draining adjustment mode in the floating process is studied. Simultaneously, a buoyancy-driven system characterization test was performed to examine the buoyancy adjustment of FUXING. When the frequency of oil draining is 15 times, the total energy consumption of FUXING is the lowest. Finally, FUXING was deployed in the northeast off the Luzon Island to validate the feasibility and reliability. The at-sea experiments indicated that the optimized oil draining adjustment mode can reduce the total energy consumption in the floating process by more than 20%. The profile data showed that the outer sea water gradually mixes with the South China Sea water after passing through the northeast off the Luzon Island.
Similar content being viewed by others
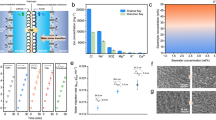
In-situ direct seawater electrolysis using floating platform in ocean with uncontrollable wave motion
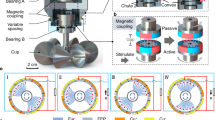
Self-powered and speed-adjustable sensor for abyssal ocean current measurements based on triboelectric nanogenerators

Flapping and powering characteristics of a flexible piezoelectric nanogenerator at Reynolds number range simulating ocean current
Introduction.
In recent decades, the observation of the deep ocean layer between 2000 and 4000 m isobaths 1 , 2 , which would help improve estimates of the ocean heat, freshwater content, and sea level rise, has become a research hotspot 1 , 2 . To meet the needs of thermal content research in deep sea water, it is necessary to extend regular 2000 m profiles to deeper depths. To develop and utilize deep oceans, deep-sea observation technology is required to provide technical support. The deep-sea self-sustaining profiling float has become an important tool for accomplishing oceanic reconnaissance and different deep-sea missions, for the sustainable development of the emerging ocean industry. This has provided tremendous economic and societal benefits 3 , 4 .
The deep-sea self-sustaining profiling float is widely applied to the ARGO project 5 , and is called a profiling float or Argo float, which dives and ascends by altering its buoyancy. As a universal platform for ocean monitoring instruments, the profiling float can take a variety of hydrological observation sensors required by the scientific research along with it, such as temperature, salinity, depth, pressure and other sensors. It can cycle vertically and send the collected ocean hydrological data underwater to the receiver on the research vessel by iridium communication. The data is transferred toward stations on land, as shown in Fig. 1 .
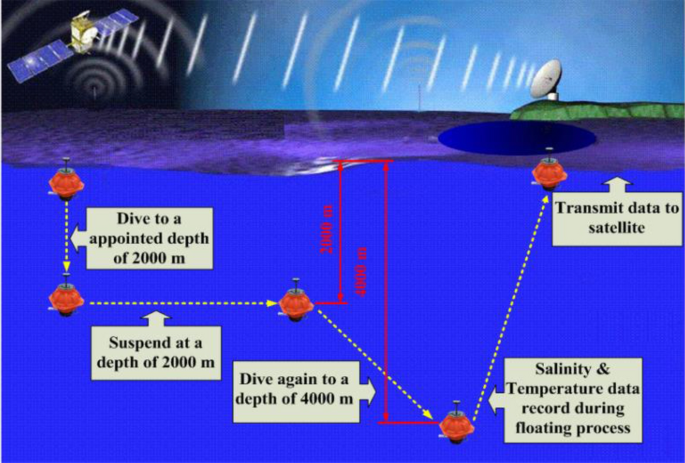
Schematic diagram of a profiling float operation cycle profile measurement.
A significant number of profiling floats have been developed to overcome engineering and scientific challenges caused by unpredictable underwater environments. The deep-sea self-sustaining profiling float, as a sophisticated artificial intelligence integration system, is a highly autonomous, self-learning unmanned platform that adapts to the volatile surroundings in complex ocean environments. Research on profiling float has become a research hotspot. Reviewing the development process of the intelligent float around the world, it has evolved from a neutral float proposed by Swollow to a currently utilized intelligent float 6 . Simultaneously, Stommel et al. proposed the concept of a neutral buoy to directly measure deep ocean currents 7 . For the neutral buoy 8 , because the data reception was performed by the shipboard acoustic equipment, both the drift range and the deployment depth were limited by the receiving range of the acoustic signal. The SOFAR (sound fixing and ranging) buoy developed by Rossby and Webb in 1970 had a lifespan of several years, and could be tracked over a wide range. However, the SOFAR buoy was bulky and huge, which brought significant inconvenience to the layout of equipment 7 , 8 , 9 . To improve the means of dumping heavy loads into the ocean in the ascending process, Davis and Webb launched the development of the autonomous Lagrangian circulation explorer (ALACE) in 1992 10 , 11 . The mature and successful application of alace buoy technology has enabled the United States, France, China and other countries to produce their own types of profile buoys for marine hydrological observation at a depth of 0~2000 m. Among the profiling floats currently operating in the global ocean real-time observation network, PROVOR 12 , ARVOR 1 , COPEX, and HM-2000 13 profiling floats have been deployed in various sea areas worldwide for several years. At present, several major profiling floats have gradually been the main carrier to work under the deep-sea, such as Deep NINJA 14 , Deep Arvor 1 , Deep SOLO 18 and Deep APEX 5 . Deep NINJA is mainly suitable for observing special environmental sea areas such as the tropical and high-latitude ice-covered seas. However, when Deep NINJA works in a sea ice environment for a long time, its buoyancy-driven system is prone to failure, resulting in a high loss probability of the profiling float. The self-ballast and lightweight characteristics of the ARVOR are maintained for the Deep Arvor. However, when the depth of Deep Arvor is greater than 2000 m, the preliminary stop challenge of the data transmission occurs owing to the deviation of the salinity value in the range of 0.01~0.02. Two types of deep floats with a spherical glass hull (Deep SOLO and Deep APEX) have been available since around 2015, which is described in the report of Zilberman and Maze (2015) 5 . But, the circuit hardware designed by Deep APEX was unstable and needs to be further optimized 17 . Compared with the hydrological data collected by SBE 9plus CTD sensor on board, the salinity profile data collected by Deep SOLO in the first 8 cycles had a certain salinity deviation (0.01~0.02 PSU pss-78) 18 . Furthermore, the Deep Arvor and Deep SOLO only met the specification of 31~32 cycles at 4000 m depth with CTD measurements. The drift at the parking depth interval was a bias of ± 50 m and ± 80 m for the Deep Arvor and Deep SOLO 16 , 18 . Data collection accuracy and profile work stability were the same for Deep APEX and Deep SOLO, as were Deep Arvor and Deep NINJA 15 , 16 , 17 , 18 . Thus, the data collection accuracy and profile operation stability of Deep Arvor and Deep SOLO need to be optimized. The major profiling float prototypes are illustrated in Fig. 2 .
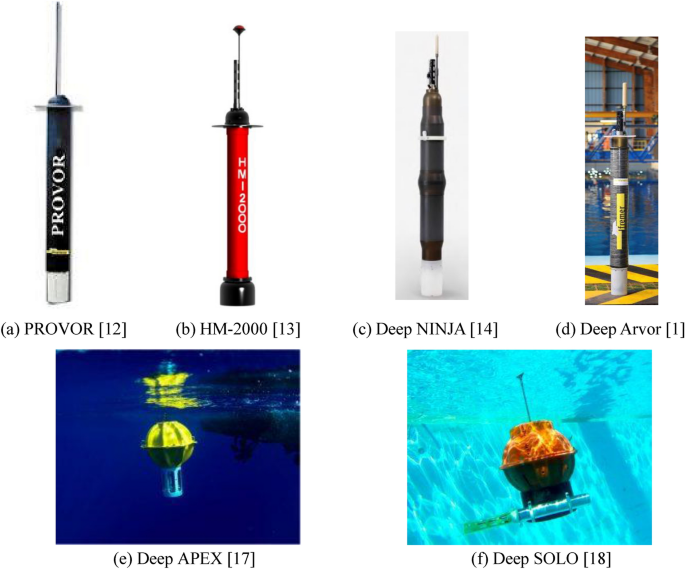
The appearance drawing of major profiling float system prototype.
A few scholars have conducted extensive research on the buoyancy-driven system for the deep-sea self-sustaining profiling float. Roemmich et al. 15 utilized a hybrid mechanism of a singlestroke to design the buoyancy-driven system for the Deep NINJA. This engine is suitable for a small vehicle operating in the deep ocean. However, the engine must pull back its piston to replenish the hydraulic oil supply after ejecting the oil. To effectively improve the buoyancy-driven engine technology of the Deep NINJA by extending its capacity to higher pressures, Le Reste et al. 16 proposed a combination of a multistroke pump and a valve to transfer oil between an external bladder. For the designed variable buoyancy engin, A high-efficiency electric motor was chosen to optimize the energy budget. However, during immersion, the hydraulic flow rate of the valve is very pressure dependent in the design scheme. Petzrick et al. 17 proposed an hydraulic buoyancy engine to realize the buoyancy-driven effect of the profiling float system. The design scheme mainly involved altering the effective volume utilized for the buoyancy adjustment using a direct current (DC) motor-driven pump to pump oil from the reservoir to the oil bladders. However, atmospheric pressure may be sufficient to overcome the force exerted by the spring on the rolling diaphragm to cause oil to flow from the oilbladders into the oil reservoir. The spring in the oil reservoir will reduce the retraction force due to the long-term use, which will prevent the piston from working properly in the oil reservoir. DAVID et al. adopted a motor-controlled high-pressure pump to let the oil flow from the polyurethane interior reservoir to the external bladder to increase buoyancy. But when the output flow of the pump suddenly increases, the flow of suction cannot meet the requirements in a short period of time, resulting in instantaneous suction and cavitation.
Although several major profiling floats have been utilized in the investigation and collection of marine environmental data, they are still in the development and testing stages. The profiling float still face several technical challenges, and stricter requirements have been proposed for their reliability. It has been developing toward multiple directions of multi-functional, great-submergence, and intelligence. However, the effective volume change of the buoyancy adjustment cannot be monitored for the buoyancy-driven mode of the profiling float during the buoyancy adjustment process 15 , 16 , 17 , 18 . In addition, the current buoyancy-driven system of the profiling float was designed to use a two-way gear pump to suction and discharge oil from the external oil bladder to achieve the depth positioning process of the profiling float 15 , 16 , 17 , 18 . More additional energy consumption would be consumed for the buoyancy-driven mode during the buoyancy adjustment process. The range of the profiling float is restricted. At present, the direct current (DC) motor-driven pump was only adopted to design the buoyancy-driven system of the profiling float 15 , 16 , 17 , 18 . But, when the inlet pressure of the bi-directional-gear pump is lower than the saturated vapor pressure of the hydraulic oil, the cavitation is easy to occur, thus reducing the volumetry efficiency of the plunger pump, the energy consumption of the profiling float is increased. In addition, the pressure housing of the profiling float still presently chooses a high-strength aluminum alloy cylindrical shell 15 , 16 . Strict requirements have been advocated for the compression resistance of the pressure housing. In this study, a Chinese profiling float named ‘FUXING’ was designed to meet the deep-ocean surveys needs at a depth of 4000 m.The pressure-proof structures of FUXING were designed in a spherical shape, and the chosen material of the spherical pressure hull was glass. The chosen material of the protective shell was polyethylene. The pressure resistance experiment was utilized to analyze the anti-pressure ability and compressibility of the FUXING hull. To increase the suction port pressure of the plunger pump and make full utilization of the spherical glass housing space, an air pump is used to provide an appropriate pressure for the internal reservoir to solve the air lock problem in the system. A combination of high-pressure plunger and air pumps was adopted to design the buoyancy-driven system. In order to ensure the buoyancy adjustment accuracy of the buoyancy-driven system, a pull line displacement sensor is used to precisely measure the piston displacement of the internal reservoir to realize the precise buoyancy regulation of FUXING. In the design process, the calculation model for the pressure change inside the spherical glass housing is provided. In order to reduce the energy consumption of FUXING operation process and improve the running time of it to a greater extent, the optimization of the oil draining adjustment mode for FUXING in the floating stage is proposed. The buoyancy regulation and continuous circulation capacity of the designed buoyancy-driven system at a depth of 0–4000 m were assessed by the hydraulic engine characterization test. Finally, the feasibility of the designed buoyancy-driven system and FUXING prototype was validated by continuous multi-profile performance tests in the northeast off the Luzon Island trials. The total energy consumption of profile work are analyzed and compared with the theoretical results. The obtained profile data also reveals the characteristics of the Tropical Water near the northeast off the Luzon Island area.
Design of the FUXING buoyancy-driven system
Buoyancy-driven system principle.
In a complex deep-sea environment, a buoyancy-driven system can take the place of a vertical propeller and play a vital role in power-free heaving motion and autonomous drifting of the FUXING. The buoyancy regulation principles of the FUXING are mainly based on altering the displacement volumes of the FUXING to control the motion, so as to measure the water temperature, salinity, depth, and other data. The buoyancy-driven system schematic of FUXING and its appearance are illustrated in Fig. 3 . 19
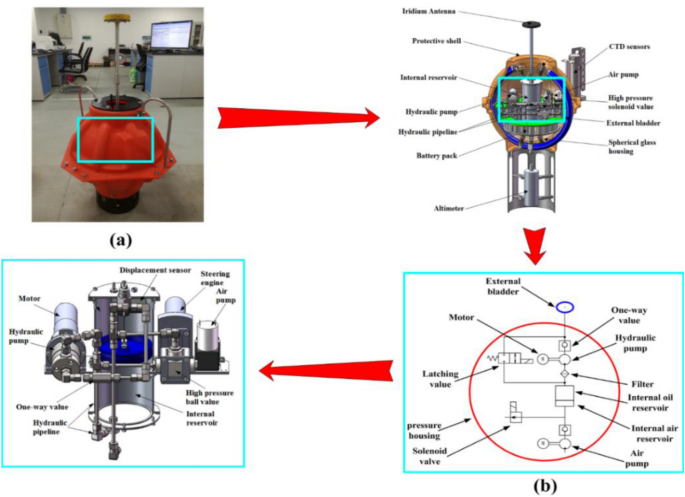
( a ) The appearance drawing of a FUXING prototype (The blue box represents the buoyancy adjustment system position of the FUXING); ( b ) Basic schematic diagram of a buoyancy adjustment system of the FUXING.
When FUXING is ready to submerge, a latching value is opened to pump the hydraulic oil of the external oil bladder into the internal oil reservoir. FUXING dives to a predefined depth because the volume of the external oil bladder becomes small to decrease the buoyancy. FUXING will be suspended in seawater for the scheduled period. When FUXING ascends, hydraulic oil flows from the internal oil reservoir through a filter to a hydraulic pump, which is driven by the motor. The single-stroke plunger pump is replaced by a smaller high-pressure plunger pump to provide ultrahigh pressure. A hydraulic pump can utilize the sphere space fully. Hydraulic oil is pumped into the external oil bladder to increase its buoyancy using a one-way value. The one-way value can prevent hydraulic oil from reversing backflow through the smaller high-pressure pressure plunger pump. The buoyancy of the FUXING begins to increase with the volume of the external oil bladder expanding. The FUXING will ascend when the buoyancy is greater than the gravity of FUXING. The vertical profile data of water temperature, pressure, and salinity will be collected by the CTD sensors during their ascent to the surface. When FUXING is pushed to the surface, the data can be transmitted via satellites. After the data are transmitted, the new dive will start to the next period until the battery is out of power 20 .
Minimum volume of internal oil reservoir for buoyancy adjustment
The relevant specifications of the FUXING listed in Table 1 .
Because the total amount of hydraulic oil in the buoyancy-driven system is known, if the amount of hydraulic oil in the internal oil reservoir is determined, the amount of hydraulic oil in the external oil bladder can be calculated. Thus, the buoyancy provided by the external oil bladder to the FUXING can be accurately measured. Therefore, the design of the internal oil reservoir component plays an important role in the design process of the entire buoyancy-driven system. To realize the compact structure and good modularization of the buoyancy-driven system, to utilize less hydraulic oil to change the larger buoyancy, and the oil quantity can be accurately adjusted; a piston-type internal oil reservoir assembly structure is designed in this study. Figure 4 illustrates the structural diagram of the internal oil reservoir, which comprises a piston, internal oil and air cavities, air and oil circuit interfaces, and a draw-wire displacement sensor. A high-precision draw-wire displacement sensor was installed in the inner oil cavity. According to the piston area S , and displacement ∆ h of the displacement sensor, the oil quantity change in the internal oil cavity can be calculated as Q = S × ∆ h . Therefore, the buoyancy adjustment can be indirectly calculated based on the amount of oil change.
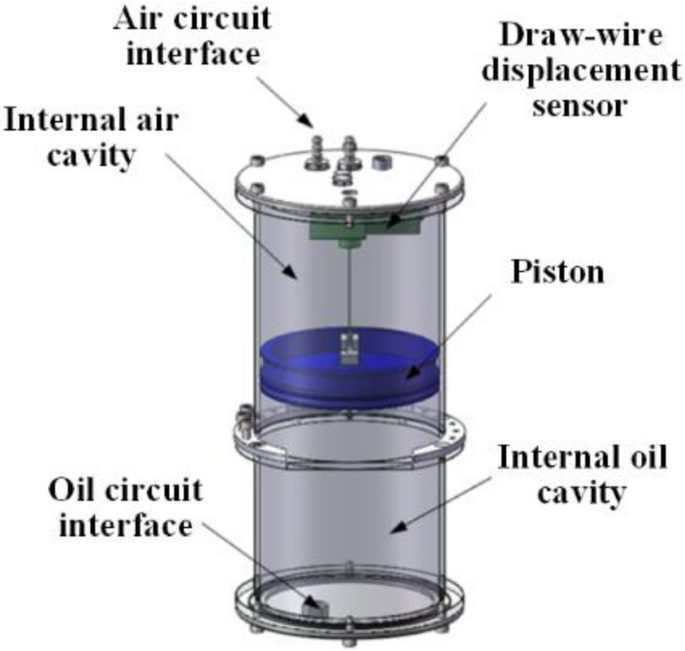
Internal structure perspective of the internal oil reservoir.
Based on the forces acting on the FUXING, combined with the FUXING hull deformation and the variation law of seawater density, the limit idea is utilized to determine the mass of the counterbalancing weight before launching the FUXING, and the volume of internal oil reservoir required for buoyancy adjustment. The force equilibrium equation of the FUXING is expressed as follows:
where F a is the resultant force of the FUXING under water; M f is the total mass of the FUXING; M Fe is the mass of the counterbalancing weight of the FUXING, g is the acceleration due to gravity, ρ is the seawater density, V f is the profiling float volume at 1 atm, V Fe is the drainage volume of the counter-balancing weight inside the FUXING, ∆V is the profiling float hull deformation caused by the underwater environment pressure and temperature, C d is the drag coefficient ( C d = 0.7), A is the projected area of the FUXING hull ( A = 0.301 m 2 ), v is the mean vertical velocity of the FUXING in both the ascending and diving processes ( v = 0.1 m/s) and Q is the hydraulic oil volume of the external oil bladder. For the convenience of analysis, the regulating oil volume of the external oil bladder is divided into two parts: one part is the neutral buoyancy regulating oil volume Q 1 related to gravity balance, and the other is the non-neutral buoyancy regulating oil volume Q 2 related to water resistance balance.
According to Eq. ( 1 ), the force equilibrium equation of the FUXING can be obtained as follows:
When the FUXING reaches its neutral buoyancy at depths of 10 m and 4000 m, the force equilibrium equation for the static condition is obtained as:
where ρ 10 and ρ 4000 are the seawater densities at depths of 10 m and 4000 m, respectively. ∆V 4000 is the FUXING hull deformation caused by the underwater environment pressure and temperature at a depth of 4000 m. It can be derived from Eq. ( 3 ) that Q 1 is mainly determined by the regulating oil volume of the external oil bladder caused by the seawater density variation and the profiling float hull deformation.
Figure 5 21 illustrates the typical hydrological data cited by Japanese scholars in the technical demonstration related to the development of the NINJA float at the 2012 ISOPE conference, corresponding to the hottest and coldest, maximum, and minimum surface densities, respectively. It can be utilized as a reference for studying the maximum variation in sea water density. In Fig. 6 , the solid and dotted lines represent the hydrological data of the Western Pacific and the North Atlantic, respectively. The limit variation range of seawater density at a water depth of 10 m to 4000 m is 1.021 × 10 3 kg/m 3 to 1.047 × 10 3 kg/m 3 .
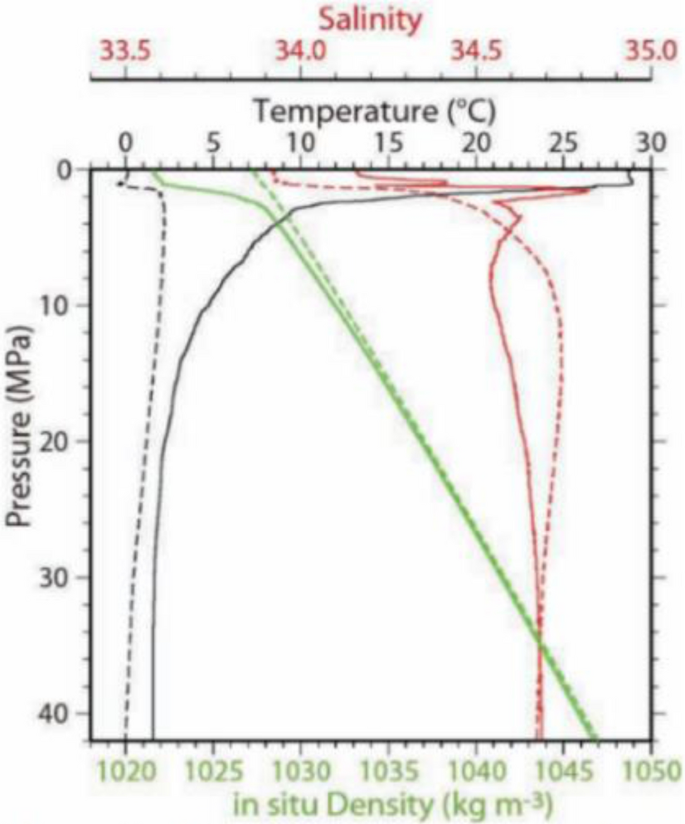
The typical hydrological data in the Western Pacific and North Atlantic by the NINJA float 21 .
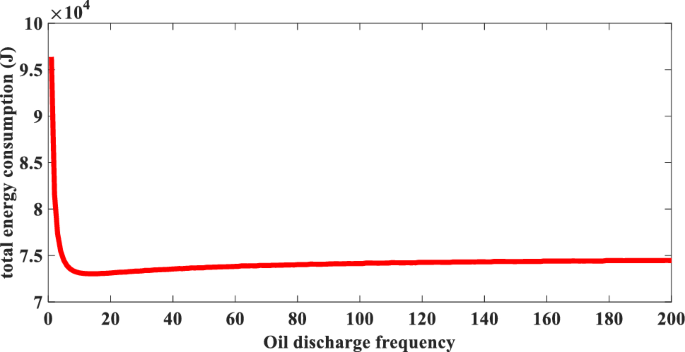
The relationship between different oil draining frequency and total energy consumption of FUXING.
According to the calculation equation of the FUXING hull deformation caused by the underwater environment pressure and temperature 19 , the FUXING hull deformation is approximately 800 ml at a depth of 4000 m. If the total mass of the FUXING is 46 kg, the counterbalancing weight is the stainless-steel weight combination of different weights, and the density of stainless steel is 7980 kg/m 3 . The counterbalancing weight of the FUXING system is calculated from Eq. ( 3 ) as 5.87 kg.
Considering that the total displacement of the designed FUXING system should not exceed 60 kg, it can be calculated from Eq. ( 3 ) that the regulating oil volume of the external oil bladder for the buoyancy adjustment is approximately 632 ml. In addition, considering the compressibility of hydraulic oil under high pressure at a water depth of 4000 m, the hydraulic oil compressibility of the external oil bladder is 1.5 × 109 Pa. The maximal adjustable oil compression under a pressure of 40 MPa is 20 ml. According to the aforementioned calculation process, the neutral buoyancy regulating oil volume Q 1 related to gravity balance is 652 ml.
The non-neutral buoyancy regulating oil volume Q 2 related to the water resistance balance is mainly utilized to regulate the upward and downward movements of the FUXING. Because the mean vertical velocity of the FUXING is approximately 0.1 m/s in both the ascending and diving processes, it can be approximated as a uniform motion. Equation ( 2 ) can be obtained as follows:
According to ( 2 ), the regulating oil volume of the external oil bladder that needs to be adjusted to stabilize the mean vertical velocity of the FUXING at 0.1 m/s is approximately 108 ml. Considering that the oil loss in the high-pressure pipeline is approximately 140 ml, the calculation results indicate that the minimum volume of the required internal oil reservoir for buoyancy adjustment is approximately 900 ml.
Analysis and calculation of air pressure variation in spherical glass housing
The end face seal was adopted between the upper and lower hemispherical shells of the spherical glass housing. It is necessary to extract a vacuum to complete the sealing of the spherical glass housing. Currently, a negative pressure state was formed in the spherical glass housing. In the working process of the buoyancy-driven system, the smaller high-pressure plunger pump has the problem of insufficient oil suction capacity, which leads to the vacuum environment, high-speed oil suction is easy to produce a vacuum chamber at the oil inlet end. That is, when the high-pressure plunger pump outputs the hydraulic oil in the internal oil reservoir placed in the negative pressure environment to the external oil bladder, it is easy to separate small vacuum bubbles at the oil inlet of the high-pressure plunger pump, resulting in air lock, which makes it difficult to output the hydraulic oil to the external oil bladder.
To solve the air lock problem and enhance the oil absorption capacity of the plunger pump, an air pump is required to pressurize the inner oil reservoir, which inevitably leads to air pressure changes inside the spherical glass housing. To ensure that the high-pressure plunger pump has a normal suction pressure during the ascending and diving processes of the FUXING, it is necessary to analyze the pressure change inside the spherical glass housing during this process.
Calculation of internal vacuum degree in the spherical glass housing
The vacuum degree is a measure of the pumped air, which means that the actual value of the system pressure is lower than the atmospheric pressure. That is, vacuum (Z) = atmospheric pressure—absolute pressure, absolute pressure = atmospheric pressure + gauge pressure (− vacuum) 22 , 23 . Before calculating the vacuum degree inside the spherical glass housing, it was necessary to determine the residual gas inside the spherical glass housing. The volume occupied by the buoyancy drive system, control system, and battery pack in the spherical glass housing was removed, and the remaining volume (V remaining volume) was the volume of residual gas. Table 2 presents the volume of each component required to calculate the remaining gas.
Given that the internal cavity diameter of the spherical glass housing is 405 mm, the maximum volume space contained in the spherical glass housing is V 1 = 0.034783 m 3 , and the remaining gas volume inside the spherical glass housing ( V remaining volume ) is calculated as follows:
The minimum volume of the internal oil reservoir is V 4 = 900 ml, the volume of the designed air bladder is V 7 = 550 ml, and the rated pressure safety factor of the external supplementary internal air chamber and the air bladder is 1.2. Then, the actual vacuum degree of the spherical glass housing ( Z′ ) is calculated as follows:
Using the aforementioned calculation, it can be observed that the internal air pressure value that guarantees the sealing of the spherical glass housing is approximately 0.56 bar.
Analysis and calculation of pressure change in spherical glass housing when diving and floating
It is known that the sealing of the spherical glass housing for the internal initial air pressure is P 1 = 0.56 bar. According to the selection of the high-pressure plunger and air pumps, the pressure of the external supplementary air bladder is P 2 = 1.2 bar, and ensuring the oil suction pressure range of the high-pressure piston pump for the normal operation of the hydraulic system is P 3 = 0.8–1.5 bar.
When the minimum suction pressure of the high-pressure plunger pump ( P 3 ) is 0.8 bar, the pre-pressure on the piston of the internal oil reservoir ( P 4 ) is:
When the minimum suction pressure of the high-pressure plunger pump ( P ′ 3 ) is 1.5 bar, the pre-pressure on the piston of the internal oil reservoir ( P ′ 4 ) is calculated as follows:
The internal gas pressure when the spherical glass housing is submerged ( P 0 ) is calculated as follows:
Whether the temperature difference between the temperature at a depth of 4000 m and the sea surface temperature affects the internal pressure change of the spherical glass housing seal during the floating process will be further discussed below.
The influence of the temperature difference between the temperature at a depth of 4000 m and the sea surface temperature is not considered.
When the minimum oil suction pressure of the high-pressure piston pump ( P 3 ) is 0.8 bar, the internal gas pressure as the spherical glass housing ascending ( P n ) is calculated as follows:
When the minimum oil suction pressure of the high-pressure piston pump ( P ′ 3 ) is 1.5 bar, the internal gas pressure as the spherical glass housing ascending ( P ′ n ) is calculated as follows:
Considering the influence of the temperature difference between the temperature at a depth of 4000 m and the sea surface temperature, it is assumed that the sea surface temperature is T 0 = 29 °C, and the temperature at a water depth of 4000 m is T n = 1.6 °C.
When the minimum oil suction pressure of the high-pressure piston pump ( P 3 ) is 0.8 bar, the internal gas pressure obtained as the spherical glass housing ascends ( P t n ) is calculated as:
When the minimum oil suction pressure of the high-pressure piston pump ( P ′ 3 ) is 1.5 bar, the internal gas pressure obtained as the spherical glass housing ascends ( P ′ t n ) is calculated as:
It can be observed from the calculation results whether the influence of the temperature difference between the temperature at a depth of 4000 m and the sea surface temperature is considered. When the oil suction pressure range of the high-pressure plunger pump is 0.8–1.5 bar, the calculation results of the internal gas pressure for the spherical glass housing ascending process meet the internal pressure requirements of the spherical glass housing seal. Furthermore, the gas pressure inside the spherical glass housing under the influence of the seawater temperature difference was lower than that without, considering the influence of the seawater temperature difference. The required parameters and numerical calculation results are presented in Table 3 .
Analysis and optimization of energy consumption
The energy consumption of single profile motion has a decisive influence on the endurance of FUXING system. Thus, the energy budget estimation for FUXING is analyzed, and the power consumption of four parts including the buoyancy-driven system, the control system, the satellite communication system and the CTD sensor during the movement of a profile is mainly studied in this paper. According to the working voltage, current and working time of the aforementioned energy-consuming units, the energy consumption required for a single profile at a depth of 4000 m can be calculated. The specific energy consumption of each unit is shown in Table 4 .
In the aforementioned energy consumption units, the main energy consumption unit of FUXING is buoyancy-driven system. Thus, the key issue to reduce the energy consumption of each profile is to optimize the energy consumption of the buoyancy-driven system. According to the analysis of the working process of FUXING, it is found that the power of the floating process is much greater than that of the diving process. In the ascent process of the profiling float, the total amount of hydraulic oil of the internal oil reservoir can be transferred to the external oil bladder by driving the motor at one time. However, to make full use of the inertial motion of the profiling float in the water and achieve the purpose of energy saving, the required oil draining amount of the profiling float is completed at different frequency by intermittently starting the motor in the floating process. For the floating process, the energy consumption of the buoyancy-driven system at different frequency of the oil draining and traditional one-time oil draining oil is analyzed in this paper.
According to the selection of the high-pressure piston pump, the approximate representation of the relationship between the input torque of the high-pressure piston pump ( T 1 ) and the seawater pressure ( p ) can be determined as 24 .
The relationship between starting power consumption of DC motor ( W s ) and its load torque ( T 2 ) is expressed as 24 .
The relationship between the motor stable operating power ( P e ) and its load torque ( T 2 ) is expressed as 33
In order to analyze the influence of the oil draining frequency of the buoyancy-driven system on the total energy consumption, the method for multiple quantitative oil draining of the buoyancy-driven system is explored to study the energy consumption optimization of FUXING during the floating process. If the frequency of oil draining of the buoyancy-driven system is ( n ) during the ascent of FUXING, and the minimum volume of the internal oil reservoir is 900 ml as the total oil draining, then the oil volume obtained by the current i -th adjustment of the external oil bladder through the buoyancy-driven system ( v ) is expressed as:
When the FUXING is in a neutral buoyancy state, the FUXING remains suspended, and the following relationship condition is satisfied:
where , V f is the FUXING volume at 1 atm; ∆V is the FUXING hull deformation caused by the underwater environment pressure and temperature ( \(\Delta V = 1.844 \times 10^{ - 11} \rho gz\) 19 ); m is the total mass of FUXING; g is the acceleration due to gravity; ρ ( z ) is the seawater density at the seawater depth z. Due to the fitting formula of the seawater density in the South China Sea is expressed as 19 .
From Eq. ( 19 ), the expression of the depth z can be calculated as follows:
According to Eqs. ( 17 ), ( 18 ) and ( 19 ), the input torque of the high-pressure plunger pump during the i th adjustment process ( T i ) is expressed as:
From the single oil draining of the high-pressure plunger pump and the displacement parameters of the pump, the working time of the DC motor corresponding to different oil draining frequency ( t ) can be expressed as follows:
Then, according to Eqs. ( 15 ) and ( 16 ), the power consumption of each adjustment of the DC motor ( W i ) is expressed as:
Thus, the total power consumption of FUXING buoyancy-driven system during the floating process ( W ) is expressed as:
It can be found from Table 4 that the main energy consumption units of FUXING are mainly concentrated in three parts: the buoyancy-driven system, the control system and the CTD sensor. The energy consumption of the buoyancy-driven system changes with the increasing of the oil draining frequency, but the increased operating time causes the energy consumption of the control system to increase. Combining with the operating energy consumption of the control system, the CTD sensor and the total power consumption of the buoyancy-driven system during the floating process, the relationship between different oil draining frequency and the total energy consumption of FUXING was obtained through the reliability test, as shown in Fig. 6 . It can be seen from Fig. 6 that the relationship between the oil drainage frequency of the buoyancy-driven system and the total energy consumption of FUXING is nonlinear. With the increasing of the oil drainage frequency of the buoyancy-driven system, the total energy consumption of FUXING first decreases and then slowly increases. When the frequency of the oil draining is one time, the total energy consumption of FUXING is 96.4 kJ, and the total energy consumption is the maximum value at this frequency. When the frequency of the oil draining is 15 times, the total energy consumption of FUXING is 73 kJ, and the total energy consumption is the lowest at this frequency. Compared with the required energy consumption value to discharge the total amount in the internal oil reservoir at one time, 24.2% energy consumption is saved.
Laboratory tests
The reliability test of the buoyancy-driven system and the pressure-proof test of the FUXING were performed before the FUXING was deployed in the the Northeast off the Luzon Island.
Reliability test of the buoyancy-driven system
In a complex deep-sea environment, the buoyancy-driven system can replace the vertical propeller and play a vital role in the power-free heaving motion and depth control of the FUXING. Thus, it is necessary to conduct a reliability test of the buoyancy-driven system. The flow rate and supply current of the buoyancy-driven system were assessed in this study. The energy budgets versus operating pressures were calculated. Moreover, the buoyancy-driven system was checked for any leakage or abnormal deformation under high pressure 25 , 26 , 27 , 28 .
The average temperatures of the sea surface and 4000 m in the Northeast off the Luzon Island are approximately 25 °C and 2 °C, respectively. The working state of the buoyancy-driven system could not be determined at 2 °C and 25 °C. In this study, the working state of the buoyancy-driven system at 2 °C and 25 °C was analyzed. A reliability test schematic of the buoyancy-driven system is illustrated in Fig. 7 . The buoyancy-driven system was powered by an external power supply. The external bladder is removed, the installation interface of the external bladder is blocked by the tooling, and peripheral equipment such as an overflow valve, pressure gauge, unloading valve, and multimeter are connected. The control program is started to run the buoyancy-driven system according to the given debugging process. The high-pressure plunger pump was activated, and the volume of the transferred oil was measured by weighing the internal oil reservoir. The overflow pressure of the overflow valve was adjusted to 5 MPa. The entire overflow pressure range of the overflow valve was 0 MPa–40 MPa with a pressure gradient of 5 MPa. When the pressure reached the preset pressure value, the preset pressure value was maintained for 5 min. The maximum and minimum values of the multimeter versus pressure were recorded during this process.
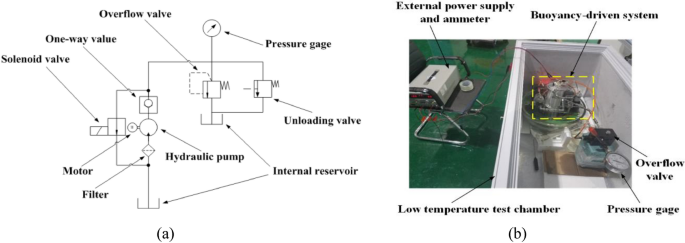
Hydraulic reliability test used for the assessment of the buoyancy-driven system efficiency: ( a ) Hydraulic reliability test used for the assessment schematic of the buoyancy-driven system; ( b ) Hydraulic reliability test photo used for the assessment schematic of the buoyancy-driven system at 2 ℃
Figure 8 a,c illustrate the variation curve of the supply current under various pressure from 0 to 40 MPa at 25 °C and 2 °C, respectively. The red and blue solid lines represent the maximum and minimum supply current variation curves of the buoyancy-driven system under various pressure from 0 to 40 MPa, respectively. Figure 8 b,d illustrate the variation curve of the flow rate under various pressure from 0 to 40 MPa at 25 °C and 2 °C, respectively. According to the reliability test results of the buoyancy-driven system, it can be observed that the supply current increases linearly with increasing pressure when the buoyancy-driven system runs at 2 °C or 25 °C. The flow rate of the buoyancy-driven system decreased with increasing pressure at 2 °C or 25 °C. Moreover, when the buoyancy-driven system runs at 2 °C or 25 °C the maximum supply current may not be over 5.5 A, and the flow rate may not be less than 45 ml/min. The buoyancy-driven system has no leakage or abnormal deformation under various pressures from 0 to 40 MPa at 2 °C or 25 °C. Because the tested maximum working pressure is 40 Mpa, the buoyancy-driven system has the ability to absorb and drain oil at a depth of 4000 m during the oil transfer process. The design requirements of the buoyancy-driven system were verified.
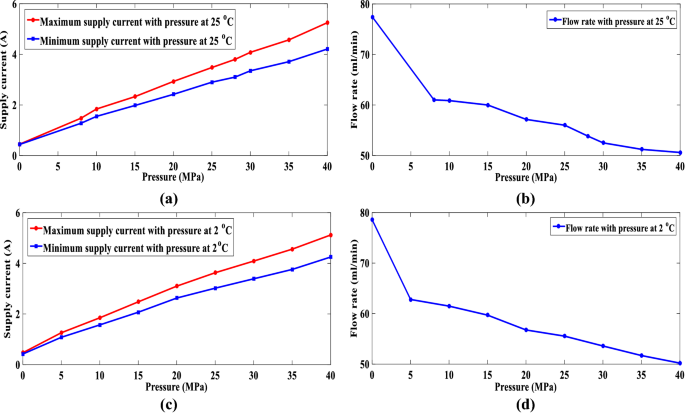
Maximum and minimum current and flow rate absorbed by the pump during oil transfers under different pressure: ( a ) Variation curve of the supply current absorbed by the pump during oil transfers with pressure at 25 °C; ( b ) Variation curve of the flow rate absorbed by the pump during oil transfers with pressure at 2 °C; ( c ) Variation curve of the supply current absorbed by the pump during oil transfers with pressure at 2 °C; ( d ) Variation curve of the flow rate absorbed by the pump during oil transfers with pressure at 2 °C.
Furthermore, Fig. 9 illustrates the comparative measurement of the theoretical power, actual efficiency, and mechanical efficiency changes under various pressures when the buoyancy-driven system is operated at 2 °C and 25 °C. The standard power, actual power, and mechanical efficiency of the buoyancy-driven system tended to increase with increasing pressure, and the maximum power consumption of entire system did not exceed 115 W.
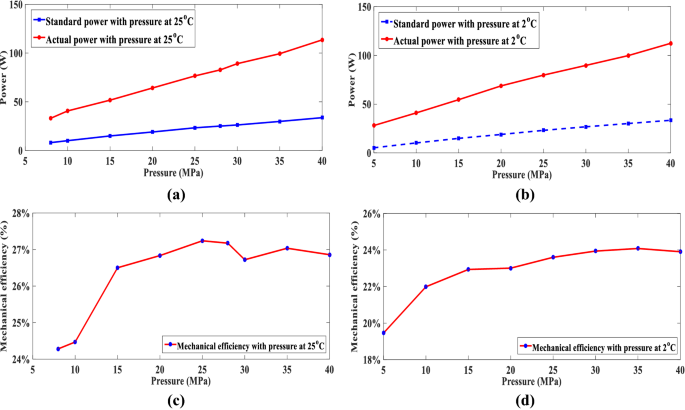
Standard power, actual power and mechanical efficiency absorbed by the pump during oil transfers under different pressure: ( a ) Variation curve of the standard power and actual power absorbed by the pump during oil transfers with pressure at 25 °C; ( b ) Variation curve of the standard power and actual power absorbed by the pump during oil transfers with pressure at 2 °C; ( c ) Variation curve of the mechanical efficiency absorbed by the pump during oil transfers with pressure at 25 °C; ( d ) Variation curve of the mechanical efficiency absorbed by the pump during oil transfers with pressure at 2 °C.
Pressure testing of the full FUXING prototypes
Pressure testing is a critical part of preparation, and a key to success in sea experiments. The purpose of the test is to check the ability of the FUXING to withstand the maximum operating pressure in the pressure testing vessel. A schematic of the pressure testing vessel system is in Fig. 10 . The experimental system of the pressure testing vessel includes a fixed frame, video camera, chain, FUXING, and video monitoring, which were placed inside the pressure testing vessel. The video monitor was placed outside the pressure-testing vessel. FUXING was placed inside the fixed frame, and the chain is connected to its bottom. The fixed frame not only protects FUXING, but also serves as a platform for the connection of the video camera. FUXING is equipped with a CTD sensor. The temperature and pressure were monitored by CTD sensors in the pressure-testing vessel. Two different tests were performed in the pressure-testing vessel system. The first was aimed at the compression of FUXING hull at a predefined depth. The purpose of the second test was to check the ability of FUXING to withstand maximum operating pressure.
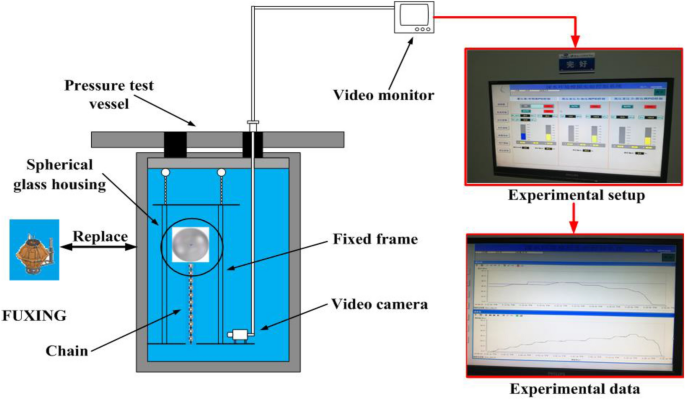
Schematic of the pressure testing vessel system: (The red box represents the experimental pressure setup and data display of the pressure testing vessel system).
A method is utilized to analyze the compression of FUXING hull at a given pressure, which lifts a chain in the pressure-testing vessel. The hull compression on FUXING with or without protective shells was simulated using a pressure testing vessel under the actual environmental conditions of deployment. The pressure testing vessel was filled with normal tap water in the experiment, and S = 0. The normal tap water temperature of the pressure-testing vessel system was measured to 25.425 °C. The normal tap water temperature remained constant during the experimental process. The effect of water temperature on the change in FUXING volume can be ignored. The water temperature was approximated as a constant. The change in the water density caused by the increasing external seawater pressure was only considered in this study. The precision of the temperature and pressure measurements was 0.003 °C and 0.04 MPa, respectively. The chain was composed of 37 weights. Each weight was 10 g. The total weight of the chain was 370 g, and its approximate volume was 32.7 ml at 1 atm. This is because the buoyancy of the chain is caused by the variation in the chain volume during pressurization. Its effect on the buoyancy change of FUXING in the water cannot be ignored during pressurization. The actual value of the lifted chain mass is the difference between the experimental and the buoyancy values of the lifted chain in this study.
At the beginning of the pressure testing experiment, the pressure-testing vessel is filled with normal tap water. FUXING with protective shells is rested on the base of the fixed frame inside the pressure test vessel, which is then sealed off. (refer to Fig. 11 ).The maximum test static pressure of the “maximum diving depth” is set to a pressure equivalent to 4000 m. Pressure is added with a pressure incremental step of 2 MPa. When the pressure reaches the preset pressure value, the dwell time is 5 min. This is because the compression extent of the FUXING volume caused by the increased pressure is less than that of the surrounding water during pressurization. FUXING begins to ascend when its gravity is less than the buoyancy. The excessive buoyancy lifts the chain attached to the bottom of the FUXING. According to reference 29 , 30 , the compression of FUXING with protective shells is calculated by the weight of the lifted chain under the.
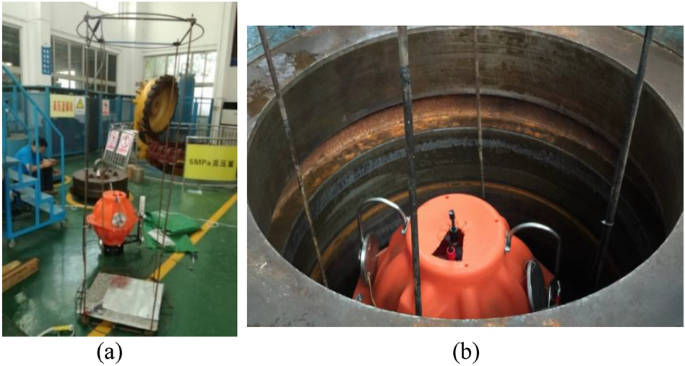
Operational mission in the pressure test vessel system: ( a ) experimental preparation process; ( b ) sealing process of the pressure test vessel system.
Subsequently, the pressure gradually decreases. After the experiment, the FUXING with protective shells is removed, and the pressure hull confirmed that there is no seal leak or abnormal deformation. Pressure testing with the measured CTD data acquisition can assure the actual results of the normal tap water density as intended. The hydrostatic proof test of FUXING with protective shells is validated against the effects of external pressure. Protective shells of FUXING are removed. The chain is connected to the bottom of the spherical glass housing. The spherical glass housing is placed in the water of the pressure test vessel. The pressure testing of the spherical glass housing is s repeated according to the above experimental procedure. The pressure incremental step and holding time at pressure during pressurization are the same as in the above experimental process. Similarly, the compression of FUXING without protective shells is calculated by the weight of the lifted chain under the corresponding pressure during pressurization.
Pressure testing makes sense to obtain the compression of FUXING hull at various external pressures. To compare the compression between FUXING with and without protective shells, the compression experimental results of FUXING shell and spherical glass housing are obtained by the pressure test vessel system, as illustrated in Fig. 12 . It can be determined that the ability of FUXING is checked to withstand a maximum operating pressure of 40 MPa. In addition, the experimental results indicate that the compression of FUXING shell is mainly determined by the compression of the spherical glass housing. To study the difference in compressibility between the water and the FUXING hull, the linear regression curve of the change rate in the water density and the FUXING hull compression with pressure is compared in Fig. 13 . It can be observed that under a pressure of 40 MPa compared to 23 MPa, the increase rate of water density is 0.715%, the compression rate of the FUXING shell is 0.51%, and the compression rate of the spherical glass housing was 0.49%. Thus, the compressibility of FUXING with protective shells is closer to that of water than that of FUXING without protective shells. If only the pressure effect is considered, when the compressibility of FUXING approximately coincides with water, FUXING will achieve a state of neutral buoyancy at any specified depth. The compressibility and sealability of FUXING hull met the requirements of the maximum diving depth.
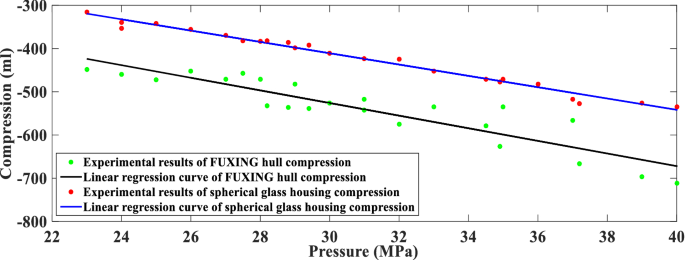
Compression linear regression curve of the FUXING hull and spherical glass housing under various pressure levels.
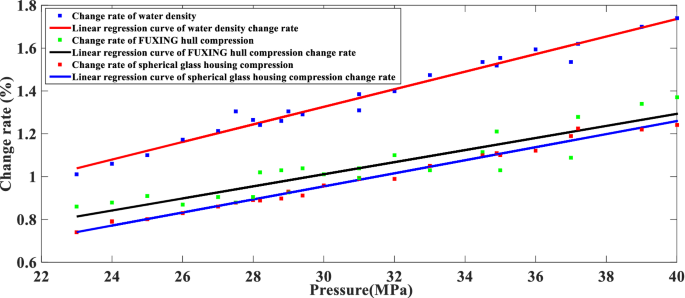
Linear regression curve of the change rate in the water density and FUXING hull compression under various pressure levels.
At-sea experimental results
A complete prototype system of FUXING was successful at sea exposure. FUXING was deployed in the Pacific Ocean, northeast off the Luzon Island, Philippines (19.08° N, 124.66° E) in August 2020, returning scientific data from depths of over 4041 m. The FUXING was equipped with a CTD sensor (Sea-Bird Electronics, Inc., SBE61) to measure the depth, conductivity and temperature. Relevant parameters of the CTD sensor are shown in Table 5 . FUXING was remotely controlled and programmed cyclically to incrementally increase its depth. Pre-programmed missions were performed to collect profiling time-series data, and to test the reliability and overall float performance for longer missions. After 48 cycles, the batteries were almost flat. A drift track from an initial test with FUXING was deployed in a dynamic area of the the Pacific Ocean, northeast off the Luzon Island, Philippines (19.08° N,124.66° E) (see Fig. 14 ). The trajectory of the FUXING with the deployment site was marked by a dot (refer to Fig. 15 ). The FUXING drifted in a southwest direction, and then turned south.
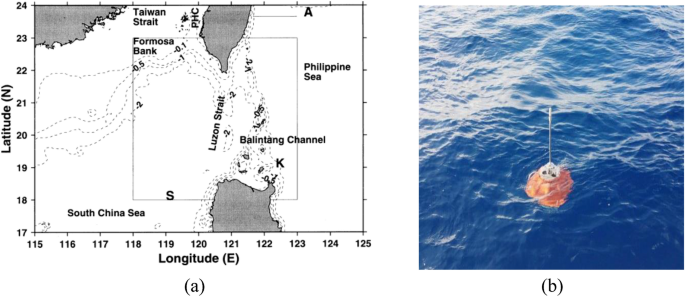
At-sea experiments of the FUXING: ( a ) Position of the FUXING deployed in the Pacific Ocean, northeast off the Luzon Island, Philippines (19.08° N, 124.66° E); ( b ) The complete prototype system of FUXING.
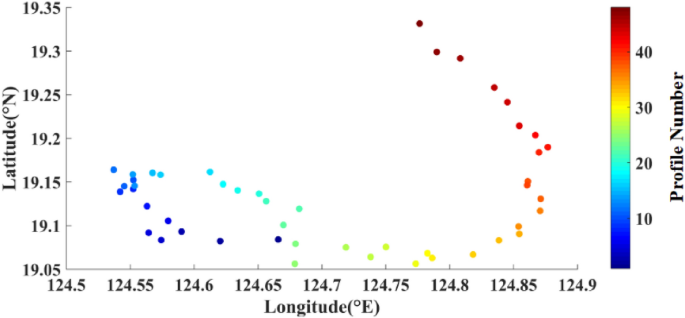
Drift track of the FUXING deployed in the Pacific Ocean, northeast off the Luzon Island, Philippines (19.08° N, 124.66° E).
FUXING dove to over 4041 m for the first time in the Pacific Ocean, northeast off the Luzon Island, Philippines. This was the first step in our technological development. Figure 16 illustrates the maximum depth and profile number obtained by FUXING during tests in August 2020, with pressure measurements starting at approximately 4041 dbar. The reproducibility of FUXING behavior mainly includes the regularity of the temporal cycles, the stability of the FUXING at parking depth, and the quality of data transmission. The regularity of the temporal cycles and the stability of FUXING at parking depth indicated good reproducibility. The ascending profile was performed at a speed of 0.09 m/s with a standard deviation of 0.005 m/s. In addition, the assessment of the designed buoyancy-driven system proved that the objective of 48 cycles at 4000 m was realistic. The drift at the parking depth interval ( \(\pm\) 41 m) was particularly stable. In order to verify the effectiveness of the optimization of the oil draining adjustment mode, the total energy consumption of the profile motion was collected before and after the optimization. Before the optimization of the energy consumption in the floating stage, the trial energy consumption showed that the float requires around 101.7 kJ for a 4000 m profile observation. Using the oil draining adjustment mode in the floating process, a total energy consumption of around 78.8 kJ was achieved for a typical 4000 m profile.
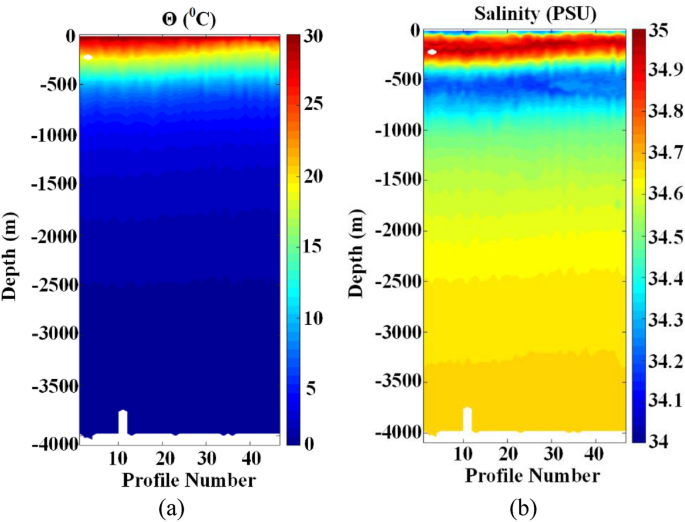
Seawater temperature, salinity and depth data from sea trials: ( a ) Relationship between seawater temperature and seawater depth; ( b ) Relationship between seawater salinity and seawater depth.
Approximately 12 buoyancy-driven system actions were needed between 4000 m and the surface. The temperature and salinity profiles collected by FUXING (displayed in Fig. 16 ) indicated an overall good quality of the transmitted data. The good quality and stability of the satellite telemetry performances were verified. It can be observed that the temperature distribution tended to decrease from the surface layer (> 20 °C) to the deep layer (< 3 °C), the upper layer isotherms were densely distributed, and the lower layer was sparse. The densest isotherms occurred at a depth of 100–500 m, and the isotherms were undulating. The temperature gradually decreased from 30 °C in the surface layer to approximately 10 °C in the vicinity of the 500 m layer, and the temperature below 500 m layer continued to decrease. It can be observed that the salinity observed by FUXING indicated a two-low and one-high distribution trend from the surface to the bottom. The first relatively low salt zone (approximately 34.40 PSU on average) appeared in the surface layer, while the second (34.15–34.30 PSU) was approximately the 700 m layer. The relatively high-salt area was near the water depth of 300 m, and its value was between 34.80 PSU and 35.10 PSU.
The temperature-salinity distribution curve of the FUXING (refer to Fig. 17 ) illustrated a "horse tail" shape, that is, the top was thick and the bottom was thin. In Fig. 17 , the subsurface high temperature and high salinity inflection point temperature is about 21 °C, and the salinity is lower than 35 PSU; while the latter inflection point temperature is about 25 °C, and the salinity is higher than 34.9 PSU. In addition, the inflection point temperature of the deep low temperature sub-high brine is about 7 °C, and the salinity is higher than 34.1 PSU.
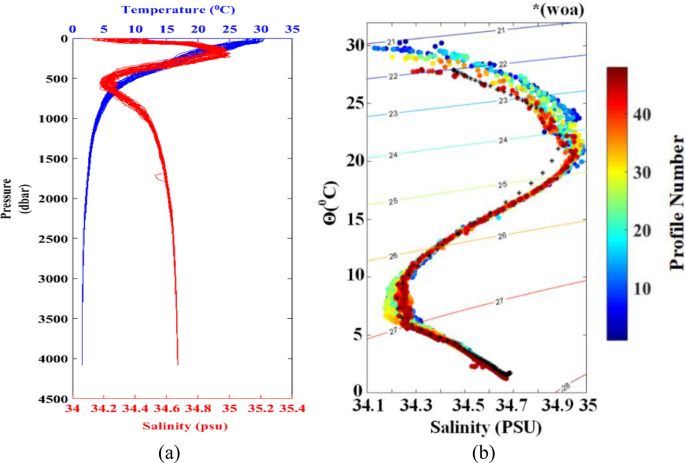
Distribution of seawater temperature and salinity in sea trials: ( a ) measured pressure versus temperature & salinity from 4000 m; ( b ) θ-S point cluster diagram of the FUXING.
As illustrated in Fig. 18 , the collected temperature data were consistent with the historical data of WOA2018 31 , 32 . The collected salinity data by FUXING was approximately a salinity bias of 0.008~0.009PSU. The salinity accuracy requirements of 0.01PSU was fully met by the international Argo plan. As with the vertical distribution of temperature, the upper salinity curve was more dispersed, and the salinity changed less as the FUXING deepened. Tests at sea are still ongoing. The reproducibility of FUXING behavior needs to be tested.
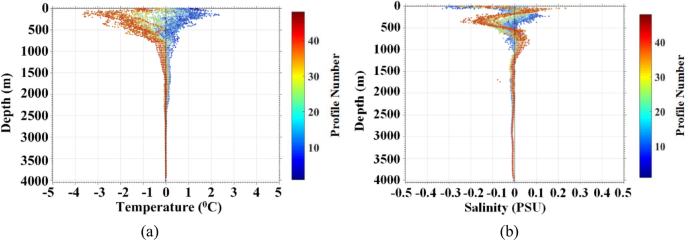
Error analysis of seawater temperature and salinity in sea trials: ( a ) error analysis of seawater temperature; ( b ) error analysis of sea water salinity.
In order to improve the ability of the profile observation in the deep ocean below 2000 m, a profile buoy named FUXING was studied, and the buoyancy-driven system was designed. The current buoyancy-driven system has problems such as air lock, high energy consumption and failure to achieve accurate buoyancy regulation. To make the designed buoyancy-driven system complete the accurate buoyancy adjustment, a draw-wire displacement sensor was adopted to measure the oil quantity of the internal reservoir to realize the precise buoyancy regulation. The combination of the high-pressure plunger pump and air pump was adopted for the designed buoyancy-driven system, and was placed in the spherical glass housing under the negative pressure. In order to solve the problem of air lock, the air pump was used to supplement air pressure to the internal oil reservoir at regular intervals to improve the oil pumping capacity of the high-pressure plunger pump to the external oil bladder. The calculation model of the pressure change inside the spherical glass housing when it is submerged and floated was given. Considering the temperature difference between the water depth of 4000 m and the sea surface, when the minimum oil absorption pressure of the high-pressure piston pump is 0.8 bar, the internal gas pressure of the spherical glass housing is 0.517 bar when it floats. When the maximum oil absorption pressure of the high-pressure piston pump is 1.5 bar, the internal gas pressure of the spherical glass housing is 0.488 bar when it floats. In order to reduce the energy consumption of FUXING and extend its service life, by introducing the external seawater pressure as the driving force, the low power consumption diving process of FUXING was effectively solved. The oil draining optimization adjustment mode the FUXING’s floating process was established by combining the dynamic model of FUXING. The reliability test of the buoyancy-driven system showed that when the frequency of the oil draining was one time and 15 times, respectively, the total energy consumption of FUXING was reduced from 96.4 to 73 kJ. The total energy consumption saving was about 24.2%. The tests at sea of FUXING revealed that the total energy consumption for one-time oil draining was 101.7 kJ, compared with 78.8 kJ for a 15 times oil draining. The optimized oil draining adjustment mode saves 22.5% of the total energy consumption. The optimization effect of the oil draining adjustment mode was verified.
In order to study the mixing characteristics of the surface water masses in the northeast off the Luzon Island, the collected temperature, salinity and depth data in the sea trials were effectively analyzed. The profile data from sea trials showed that the water structure in the northeast off the Luzon Island area was characterized by the high temperature and low salinity in the surface layer, high temperature and high salinity in the subsurface layer, low temperature and low salinity in the middle layer, and low temperature and sub-high salinity in the deep layer. The dispersion of the temperature and salt point aggregation in the surface, subsurface and middle water bodies reflected the mixing intensity of water bodies in this layer. In the θ-S point cluster diagram, all the dots (salinity maximum data) ranged between 34.1PSU and 35PSU. Because the θ-S point cluster values of the anomalously high-salinity water were between the mean θ-S point cluster values in the northern South China Sea and north Pacific subsurface water 33 . The results as mentioned above showed that the outer sea water gradually mixes with the South China Sea water after passing through the northeast off the Luzon Island, implying that the subsurface waters share characteristics with the North Pacific Tropical Water. Therefore, it is of scientific and practical value to investigate the changes in the Kuroshio intrusion and the hydrological cycle characteristics of the Tropical Water.
To extend the deep ocean observation depth of 2000 m down to 4000 m, this study presented a novel buoyancy-driven self-sustaining profile float that had been applied to ocean observations in the northeast off the Luzon Island. For the buoyancy-driven system, it was proposed to utilize a draw-wire displacement sensor to accurately measure the displacement of the piston in the inner oil reservoir to realize the precise buoyancy adjustment of FUXING. Simultaneously, an air pump was utilized to provide an appropriate pressure for the internal oil reservoir to solve the air lock problem in the system. To ensure the oil pumping capacity of the high-pressure plunger pump, the variation in gas pressure inside the spherical glass housing under the maximum and minimum oil suction pressures of the high-pressure piston pump were discussed. To reduce the energy consumption of the buoy operation process, the optimized oil draining adjustment mode in the floating process was proposed. It is found that the total energy consumption of FUXING is nonlinear with the oil draining frequency. When the floating process is adjusted 15 times for a buoyancy, the energy consumption is the lowest. A reliable buoyancy adjustment cycle was completed for the designed buoyancy-driven system under various pressures of 0–40 MPa at 2 °C or 25 °C in the hydraulic engine characterization test. The anti-pressure performance of the designed FUXING was checked by a pressure resistance test to withstand the maximum operating pressure of 40 MPa. Longer missions were planned to be conducted in the northeast off the Luzon Island in August 2020, where the overall FUXING performance would be initially tested. The results of the at-sea experiments indicated that the CTD sensor carried by the designed FUXING in the sea trials realized a maximum working depth of 4041 m and the requirement for continuous measurement of 48 profiles. The obtained temperature, salinity, and depth data had an overall good quality of hydrological data acquisition and transmission, thus, verifying the stability of the FUXING to carry multiple sensors and continuous hydrology measurement of multiple profiles. The results showed that the optimized oil draining adjustment mode can be used as the profiling float’s buoyancy regulation strategy to optimize the energy consumption of FUXING. The mixing characteristics of the external sea water mass passing through the northeast sea area of Luzon Island are effectively observed by the collected hydrological data. It is found that the outer sea water gradually mixes with the South China Sea water after passing through the northeast off the Luzon Island. The characteristics and distribution of the Tropical Water near the northeast off the Luzon Island area will be further analyzed.
Smith, R. N. & Huynh, V. T. Controlling buoyancy-driven profiling floats for applications in ocean observation. IEEE J. Ocean. Eng. 39 (3), 571–586 (2014).
Article ADS Google Scholar
Liu, G. et al. Dynamics modeling and control simulation of an autonomous underwater vehicle. J. Coast. Res. 73 , 741–746 (2015).
Article Google Scholar
D’Asaro, E. A. Performance of autonomous Lagrangian floats. J. Atmos. Ocean. Technol. 20 (20), 896–911 (2003).
Bishop, J. K., Davis, R. E. & Sherman, J. T. Robotic observations of dust-storm enhancement of carbon biomass in the north Pacific. Science 298 (5594), 817–821 (2002).
Article ADS CAS PubMed Google Scholar
Nathalie, Z. & Guillaume, M. Report on the deep Argo implementation workshop. Deep Argo Implementation Workshop . Hobart, May 5–7th, 2015.
Swallow, J. C. A neutral-buoy float for measuring deep current. Deep Sea Res. 3 (1), 74–81 (1953).
Rossby, T. & Webb, D. Observing abyssal motion by tracking Swallow floats in the SOFAR channel. Deep Sea Res. 17 (18), 359–365 (1970).
ADS Google Scholar
Rossby, T., Dorson, D. & Fontaine, J. The RAFOS system. J. Atmos. Ocean. Technol. 3 (4), 672–679 (1986).
Swift, D. D. & Riser, S. C. RAFOS floats: Defining and targeting surfaces of neutral buoyancy. J. Atmos. Ocean. Technol. 11 (4), 1079–1092 (2009).
Davis, R. E., Webb, D. C., Regier, L. A. & Dufour, J. The autonomous Lagrangian circulation explorer (ALACE). J. Atmos. Ocean. Technol. 9 (3), 264–285 (1992).
Davis, R. E., Sherman, J. T. & Dufour, J. Profiling ALACEs and other advances in autonomous subsurface floats. J. Atmos. Ocean. Technol. 18 (6), 982–993 (2001).
Gould, W. J. From swallow floats to Argo—the development of neutrally buoyant floats. Deep Sea Res. Part II 52 (3–4), 529–543 (2005).
Hanling, W. et al. Design and experimental research on deep water pressure resistance system of Argo float. J. Coast. Res. 2018 (83), 116–123 (2018).
Google Scholar
Izawa, K., et al . On the weight adjustment of profiling floats. ARGO Technical Report FY2001, Japan Marine Science and Technology Center, 18–35 (2002).
Kobayashi, T., et al . Deep NINJA: A new profiling float for deep ocean observation. In The Twenty-second International Offshore and Polar Engineering Conference , 454–461 (OnePetro, 2012).
Le Reste, S. et al. “Deep-Arvor”: A new profiling float to extend the Argo observations down to 4000-m depth. J. Atmos. Ocean. Technol. 33 (5), 1039–1055 (2016).
Petzrick, E., Truman, J. & Fargher, H. Profiling from 6,000 meter with the APEX-Deep float. In 2013 OCEANS-San Diego , 1–3 (IEEE, 2013).
Roemmich, D. et al. Deep SOLO: A full-depth profiling float for the Argo Program. J. Atmos. Ocean. Technol. 36 (10), 1967–1981 (2019).
Wang, Q., Qiu, Z., Li, H., Yang, S. & Li, X. Ballasting weight on net buoyancy changes and submergence depth for a spherical buoyancy–driven intelligent float based on the ballasting method. IEEE Access 7 , 165943–165960 (2019).
Qiu, Z., Wang, Q., Li, H., Yang, S. & Li, X. Depth control for a deep-sea self-holding intelligent buoy under ocean current disturbances based on finite-time boundedness method. IEEE ACCESS 7 , 114670–114684 (2019).
Kobayashi, T., et al . Deep NINJA: A new float for deep ocean observation developed in Japan. In 2011 IEEE Symposium on Underwater Technology and Workshop on Scientific Use of Submarine Cables and Related Technologies , 1–6 (2011).
Collins, C. A., Margolina, T. & Rago, T. A. Looping RAFOS floats in the California current system. Deep Sea Res. Part II 85 (85), 42–61 (2013).
D’Asaro, E. A. et al. A Lagrangian float. J. Atmos. Ocean. Technol. 13 (6), 1230–1246 (1996).
Mu, W. et al. Depth control method of profiling float based on an improved double PD controller. IEEE Access 7 , 43258–43268 (2019).
Bai, Y. et al. Design and depth control of a buoyancy-driven profiling float. Sensors 22 (7), 2505 (2022).
Article ADS PubMed PubMed Central Google Scholar
Elkolali, M., Al-Tawil, A. & Alcocer, A. Design and testing of a miniature variable buoyancy system for underwater vehicles. IEEE Access 10 , 42297–42308 (2022).
Veeraragavan S, Maurya S, Suresh G, et al. Optimization of Deep-sea Profiling Float based on Ballasting Methodology. OCEANS 2022-Chennai. IEEE, 2022, 1–5.
Liu, Y. et al. Research on the operational stability and energy consumption of the profiling float. J. Coast. Res. 99 (sp1), 21–30 (2020).
Article CAS Google Scholar
Pausch, S., Below, D. & Hardy, K. Under high pressure: Spherical glass flotation and instrument housings in deep ocean research. Mar. Technol. Soc. J. 43 (5), 105–109 (2009).
Reynolds, T., Lomacky, O. & Krenzke, M. Design and analysis of small submersible pressure hulls. Comput. Struct. 3 (5), 1125–1143 (1973).
Shen, H. et al. The seasonal variation of the anomalously high salinity at subsurface salinity maximum in northern south china sea from Argo data. J. Mar. Sci. Eng. 9 (2), 227 (2021).
Yu, L., et al. Revisiting the global patterns of seasonal cycle in sea surface salinity. J. Geophys. Res. Oceans 126 (1), 1-70 (2021).
Sun, Q. et al. A clustering-based approach to ocean model–data comparison around Antarctica. Ocean Sci. 17 (1), 131–145 (2021).
Download references
Acknowledgements
The authors wish to thank the State Key Laboratory of Precision Measuring Technology and Instruments for their helpful comments. This study is supported by the Wenhai Program of Qingdao National Laboratory for Marine Science and Technology (No. 2017WHZZB0101), the demonstration project of Marine Economic Innovation and Development for the National 13th Five-Year Plan (No. BHSF2017-27), the National Natural Science Foundation of China (Grant No. 11975132, No. 62001262 and No. 62001263), and the Nature Science Foundation of Shandong Province (Grant No. ZR2020QF008 and No. ZR2021QA037). Qiang Wang designed the pressure testing and wrote the paper. Xingfei Li analyzed the hydraulic engine characterization test and revised the paper. Zurong Qiu analyzed the pressure testing and revised the manuscript. Shaobo Yang analyzed the at-sea experimental data. Hongyu Li performed the at-sea experiment.
Author information
Authors and affiliations.
College of Information and Control Engineering, Qingdao University of Technology, Qingdao, 266525, China
State Key Laboratory of Precision Measuring Technology and Instruments, Tianjin University, Tianjin, 300072, China
Qiang Wang, Zurong Qiu & Xingfei Li
College of Ocean Science and Engineer, Shandong University of Science and Technology, Qingdao, 266590, China
Shaobo Yang & Hongyu Li
Qingdao National Laboratory for Marine Science and Technology, Qingdao, 266003, China
Qiang Wang, Shaobo Yang, Hongyu Li & Xingfei Li
You can also search for this author in PubMed Google Scholar
Contributions
Q.W. designed the pressure testing and wrote the paper. X.L. analyzed the hydraulic engine characterization test and revised the paper. Z.Q. analyzed the pressure testing and revised the manuscript. S.Y. analyzed the at-sea experimental data. H.L. performed the at-sea experiment. All authors reviewed the manuscript.
Corresponding author
Correspondence to Xingfei Li .
Ethics declarations
Competing interests.
The authors declare no competing interests.
Additional information
Publisher's note.
Springer Nature remains neutral with regard to jurisdictional claims in published maps and institutional affiliations.
Rights and permissions
Open Access This article is licensed under a Creative Commons Attribution 4.0 International License, which permits use, sharing, adaptation, distribution and reproduction in any medium or format, as long as you give appropriate credit to the original author(s) and the source, provide a link to the Creative Commons licence, and indicate if changes were made. The images or other third party material in this article are included in the article's Creative Commons licence, unless indicated otherwise in a credit line to the material. If material is not included in the article's Creative Commons licence and your intended use is not permitted by statutory regulation or exceeds the permitted use, you will need to obtain permission directly from the copyright holder. To view a copy of this licence, visit http://creativecommons.org/licenses/by/4.0/ .
Reprints and permissions
About this article
Cite this article.
Wang, Q., Qiu, Z., Yang, S. et al. Design and experimental research of a novel deep-sea self-sustaining profiling float for observing the northeast off the Luzon Island. Sci Rep 12 , 18885 (2022). https://doi.org/10.1038/s41598-022-23208-7
Download citation
Received : 30 March 2022
Accepted : 26 October 2022
Published : 07 November 2022
DOI : https://doi.org/10.1038/s41598-022-23208-7
Share this article
Anyone you share the following link with will be able to read this content:
Sorry, a shareable link is not currently available for this article.
Provided by the Springer Nature SharedIt content-sharing initiative
This article is cited by
Digital twins: a stepping stone to achieve ocean sustainability.
- Asaf Tzachor
- Ofir Hendel
- Catherine E. Richards
npj Ocean Sustainability (2023)
By submitting a comment you agree to abide by our Terms and Community Guidelines . If you find something abusive or that does not comply with our terms or guidelines please flag it as inappropriate.
Quick links
- Explore articles by subject
- Guide to authors
- Editorial policies
Sign up for the Nature Briefing newsletter — what matters in science, free to your inbox daily.

METHODS article
Pressure-retaining sampler and high-pressure systems to study deep-sea microbes under in situ conditions.
- 1 Aix Marseille Univ., Université de Toulon, CNRS, IRD, MIO UM 110, Marseille, France
- 2 Sorbonne Université, CNRS, Laboratoire d'Océanographie de Villefranche, LOV, Villefranche-sur-Mer, France
The pelagic realm of the dark ocean is characterized by high hydrostatic pressure, low temperature, high-inorganic nutrients, and low organic carbon concentrations. Measurements of metabolic activities of bathypelagic bacteria are often underestimated due to the technological limitations in recovering samples and maintaining them under in situ environmental conditions. Moreover, most of the pressure-retaining samplers, developed by a number of different labs, able to maintain seawater samples at in situ pressure during recovery have remained at the prototype stage, and therefore not available to the scientific community. In this paper, we will describe a ready-to-use pressure-retaining sampler, which can be adapted to use on a CTD-carousel sampler. As well as being able to recover samples under in situ high pressure (up to 60 MPa) we propose a sample processing in equi-pressure mode. Using a piloted pressure generator, we present how to perform sub-sampling and transfer of samples in equi-pressure mode to obtain replicates and perform hyperbaric experiments safely and efficiently (with <2% pressure variability). As proof of concept, we describe a field application (prokaryotic activity measurements and incubation experiment) with samples collected at 3,000m-depth in the Mediterranean Sea. Sampling, sub-sampling, transfer, and incubations were performed under in situ high pressure conditions and compared to those performed following decompression and incubation at atmospheric pressure. Three successive incubations were made for each condition using direct dissolved-oxygen concentration measurements to determine the incubation times. Subsamples were collected at the end of each incubation to monitor the prokaryotic diversity, using 16S-rDNA/rRNA high-throughput sequencing. Our results demonstrated that oxygen consumption by prokaryotes is always higher under in situ conditions than after decompression and incubation at atmospheric pressure. In addition, over time, the variations in the prokaryotic community composition and structure are seen to be driven by the different experimental conditions. Finally, within samples maintained under in situ high pressure conditions, the active (16S rRNA) prokaryotic community was dominated by sequences affiliated with rare families containing piezophilic isolates, such as Oceanospirillaceae or Colwelliaceae. These results demonstrate the biological importance of maintaining in situ conditions during and after sampling in deep-sea environments.
Introduction
Only 5% of the ocean has been explored using remote instruments and <0.01% has been sampled and studied ( Ramirez-Llodra et al., 2010 ). Despite the deep sea, being the largest ecosystem of the ocean (mean depth of about 3,500 m, i.e., 35 MPa), with the greatest reservoir of microbes ( Whitman et al., 1998 ), it still hasn't been sampled or studied adequately under in situ conditions. This major issue is mainly the consequence of technical limitations that both restrict access to this environment and make sampling under in situ pressure conditions difficult.
Microorganisms living in the deep-sea are subjected to well-known conditions: high hydrostatic pressure, low temperature, high concentrations of inorganic matter, and low organic carbon content. Pressure-adapted microorganisms have been isolated from many deep-sea sites and are defined as piezophiles when their optimum growth occurs at high hydrostatic pressure. Using laboratory pressure vessels, the characteristics of piezophiles have been described, including membrane properties, motility, nutrient transport and DNA replication and translation under elevated hydrostatic pressure (see e.g., Bartlett et al., 2007 ; Lauro and Bartlett, 2008 ).
In deep-sea environments, microorganisms represent the main players in the biological carbon pump by consuming and mineralizing organic matter as it sinks down the water column ( Cho and Azam, 1988 ; Aristegui et al., 2009 ). However, one of the main biases in deep-sea metabolic activity estimations is that, most of the time, they are measured at atmospheric pressure following the decompression of the water sample. As a result of decompression, significant shifts in prokaryotic activity ( Tamburini et al., 2013 ; Wannicke et al., 2015 ), community composition ( La Cono et al., 2009 , 2015 ), and gene expression ( Edgcomb et al., 2016 ) have been identified. In a previous review ( Tamburini et al., 2013 ), we considered the hydrostatic pressure effects on deep-sea natural communities. Analysis of datasets in the literature, show that under stratified conditions, deep-sea communities are adapted to in situ conditions of high pressure, low temperature, and low organic matter. Measurements from decompressed samples that are incubated at atmospheric pressure thus underestimate in situ activities. Exceptions that can lead to overestimations can be attributed to deep sea mixing events, large influxes of surface particles, or the provision of excessive organic matter during experimentation ( Tamburini et al., 2013 ).
In order to study the deep-sea environment, it is necessary to perform in situ measurements or continuously maintain the hydrostatic pressure at in situ temperature from deep-sea water sampling through to the transfer and measurement of microorganism activity and community composition. Over the last 40 years, pressure-retaining sampler prototypes have been developed by several laboratories using different approaches, but none are available to the scientific community without local engineering expertise or high-pressure system facilities. For the oldest systems refer to Bianchi et al. (1999) , Jannasch et al. (1973) , Tabor and Colwell (1976) or for an historical review of microbial pressure-retaining samplers see Tamburini (2006) . Recently, new pressure-retaining samplers have been locally developed [without being exhaustive NIOZ, the Netherlands; JAMSTEC, Japan—( Kim and Kato, 2010 ); WHOI, USA—( McNichol et al., 2016 ); SCRIPPS, USA—( Peoples et al., 2019 )].
Here we describe a ready-to-use pressure-retaining sampler capable of collecting and maintaining samples under in situ pressure conditions (up to 60 MPa) during sampling and the ascent from ocean depth. Along with a complete high-pressure setup, we show how to perform experiments, including replicates, transfer, and subsampling, without decompression. Technical specifications and proof of concept are described with examples of data including oxygen concentration and prokaryotic diversity from incubation experiments. These latter were carried out from a deep natural prokaryotic community collected at 3,000 m-depth in Mediterranean Sea, a first series being maintained under in situ pressure compared to another series incubated at atmospheric pressure.
Materials and Methods
High-pressure bottles (hpbs).
Two kinds of High-Pressure Bottles (HPBs) are available, one in stainless-steel coated with PEEK (Poly-Ether-Ether-Ketone) described in Bianchi et al. (1999) and Tamburini et al. (2003) , and one in titanium (described below). Different volumes can also be used: 500 mL HPBs used for sampling and 50 mL HPBs used for incubation.
Titanium alloy is widely used in oceanography instrumentation as it meets corrosion constraints linked to biofouling and prolonged immersion at great depths, or those seen in hydrothermal vents such as extreme temperatures and pH. Titanium, in particular TA6V ELI grade 5 used in the medical field, has several interesting mechanical properties when working at high-pressure. The tear resistance of TA6V is 3 times higher than 316L and the elastic limit of TA6V is 4 times higher than 316L. Densities are 4.33 g cm −3 and 8 g cm −3 , respectively for TA6V ELI and 316L. Such differences facilitate manual handling. In addition, TA6V ELI, like other titanium, is resistant to corrosion, specifically to pitting and crevice corrosion.
Based on our previous experience expertise, we built a new generation of HPBs in Titanium TA6V ELI grade 5 for medical use (2686-0000, Top Industrie SAS, https://www.top-industrie.com ) avoiding the use of PEEK coating which is an expensive consumable. These bottles are similar in principle to those made in 316L stainless-steel with PEEK coating described by Bianchi et al. (1999) and Tamburini et al. (2003) ( Figure 1A ). An important additional improvement was made using a top-end cap devoted to optical measurements. Ti-HPBs are 500 mL Titanium TA6V ELI cylinders with PEEK floating-pistons fitted with two polyurethane seals as they are more suitable for longitudinal friction. Maximum operating pressure and operating temperatures are 60 MPa and 50°C. The screw top end-cap (in titanium TA6V ELI) fitted with two O-rings is composed of two parts (see details in Supplementary Image 1 ). Main part with 4 pipes, 2 screwed pipe connections 1/8″ (3.2 mm O.D.) for sample pathway, 1 blind pipe for PT100 sensor with a diameter of 6 mm and length 60 mm and 1 pipe for the optic fiber with a diameter 5 mm and a length of 82 mm. The second part is 4 mm thick, screwed into the main part with three titanium grade 5 screws to fix a sapphire window for optical measurements. The bottom end-cap (in titanium TA6V ELI) has only one connection (see in the text below).
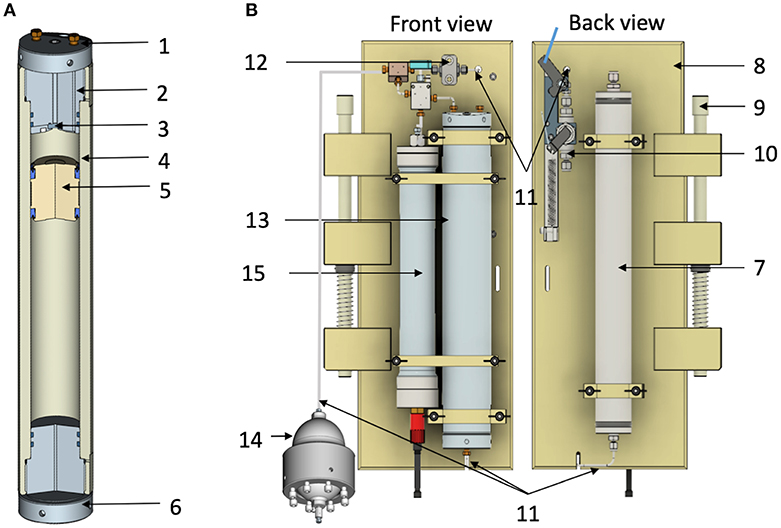
Figure 1. (A) Cross section of the Titanium TA6V 500 mL High Pressure Bottle (HPBs). (B) Schematic drawing of the front and back views of the High-Pressure Sample Unit (HPSU). 1. Top end-cap devoted to oxygen measurement; 2. 1/8″ (3.2 mm O.D.) Inlet flowthrough; 3. Sapphire window; 4. Main core; 5. PEEK floating-piston with lip seal; 6. Bottom end-cap. In the part, above floating-piston seawater sample is in contact with sapphire window (see details in the text). The part below the floating-piston contains sterile milliQ water which serve as hydraulic brakes when passing through the thin catheter into the exhaust tank (7); 8. Polypropylene main frame; 9. Push rod for attachment to a rosette system. When the (10) inlet-valve is opened by magnetically activated lanyard release, the seawater enters the hydraulic circuit through a 1/8″ (3.2 mm O.D.) stainless-steel tube (11), via one check valve (12), to fill in the HPB (13), the pressure accumulator (14), and the aero-hydraulic pressure sensor (15).
Cleaning Procedure
HPBs are washed with pressurized milliQ water using a pre-cleaned stainless-steel pressurized container. For washing HPBs, pressurized milliQ water moves the floating-piston in two ways sequentially. This backward and forward movement is repeated three times and the floating-piston is placed at the top of the HPB. Hence, the part below the floating-piston is fully filled with milliQ water. Finally, HPBs were sterilized in a pressure-sealed unit (for 20 min at 110°C). A more thorough cleaning procedure can also be used as described in Tamburini et al. (2009b) depending on the application and before starting the cruise.
High-Pressure Sampler Unit (HPSU)
To perform deep-water sampling with maintained elevated pressure, both 500 mL HPBs, described previously, are fitted onto a specific frame, designated the high-pressure sampler unit (HPSU, described in Figure 1B ). The HPSU can be fitted onto a CTD carousel beside the Niskin bottles (using the same attachment push-rod system), widely used in oceanography but providing decompressed deep-water samples.
To make it accessible to potential future users, we have standardized the manufacturing of the HPSU in collaboration with Top Industrie SAS (Vaux-le-Penil, France). The HPB is fitted by two flanges onto a polypropylene frame (287 × 600 mm) and connected to the top-part at the inlet-valve (see Supplementary Image 2 for details) with a check-valve and to the bottom-part on the exhaust tank (a stainless-steel tank, 480 mm total length, 55 mm O.D.). The Supplementary Image 2 shows the schematic drawing of the quarter-turn inlet-valve, which is set to closed position, thanks to a nylon wire (under tension using a strong spring) on the corresponding CTD-carousel trigger until fired for sampling. Moreover, each HPB is linked to the hydraulic part of autonomous data-logger pressure sensors (2903-0000, Top Industrie SAS), these are independent of the CTD carousel and gives quality control of samples before processing. HPBs are connected to an aero-hydraulic pressure accumulator (2820-1300, Top Industrie SAS) to counterbalance drops in pressure (see the description in Bianchi et al., 1999 ).
High-Pressure Sampling
For sampling, the HPSU is fitted to a CTD-carousel. The photograph in Figure 2A shows four HPSUs mounted on a 24-bottle CTD-carousel. At the desired depth, the CTD carousel trigger is fired, freeing a spring to open the inlet-valve with a quarter turn. The seawater enters the hydraulic circuit through a 1/8″ (3.2 mm O.D.) stainless-steel tube, via one check-valve, filling the HPB, the pressure accumulator and the pressure sensor. As the seawater enters the HPB, the floating-piston moves and flushes out the milliQ water below the floating-piston into the exhaust tank through a stainless-steel-tube (1/51″ I.D.−0.5 mm I.D.). This ensures a continuous and slow flow rate during the downward movement of the floating-piston and prevents damage to the floating-piston as the HPB fills up with seawater. Seawater sampling ends when the floating-piston sits on the bottom end-cap ( Figure 1B and animated drawing in Supplementary Video 1 ). The check-valve means there is no need for a closing valve or an additional trigger. Once the inlet-valve is triggered to open, the sampling time is estimated to be around 10 min to guarantee full-filling and equilibrium with the pressure accumulator.
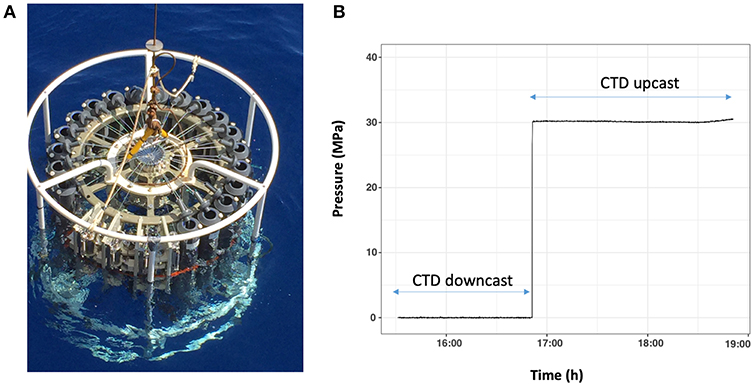
Figure 2. (A) Photograph of four HPSUs mounted on a rosette system. In this example, four HPSUs are taking the place of 5 Niskin-bottles. (B) Plot of recorded data by pressure sensor during sampling. Pressure sensor begins to record data when inlet-valve of frame is open. Data is recorded during all the casts and stored in data logger. Recorded data are available on board to check quality of collected samples.
Once the HPSU is back on board, the master pressure sensor is connected to the computer to check the monitored pressure recorded during sampling and to determine its quality (pressure variability). It can be observed that the hydrostatic pressure is maintained at ± 5% during the up-cast of the carousel. Once on board the ship, HPSUs are recovered and transferred immediately into our mobile MIO-HPLab container ( Supplementary Image 3 ). HPSUs are then placed in the in situ temperature water baths before being processed to offset the slight increase in temperature during up-cast and manual handling on deck. An example on Figure 2B shows a sample collected at a depth of 3,000 m, it can be observed that the hydrostatic pressure is maintained throughout the ascent without pressure variation.
Transfer and Subsampling in Equi-Pressure Mode
An important improvement was made in transferring the main sample and several sub-samples (obtained with the HPSU) under an equi-pressure mode maintaining at all times the high hydrostatic pressure, using a piloted pressure generator (PPG). This procedure can be used to obtain replicates of the same activity measurement.
Piloted Pressure Generator
The piloted pressure generator is a PHMP 600-600 (2493-0000, Top Industrie SA). It consists of a TA6V reservoir with a maximum operating pressure of 60 MPa, a useful capacity volume of 600 mL and a maximum flow rate of 50 mL min −1 . The PMHP 600-600 is filled with milliQ water that is compressed by a piston regulated by a brushless motor Servomotor Compax 3. This enables us to work with hydrostatic pressure safely. The PMHP 600-600 operates in three modes: pressure, volume and flow. The draining and filling/pressure input is controlled by two 1/8″ electro-valves piloted by Labview software (National Instruments™).
Protocol to Transfer and Subsample Under Equi-Pressure Mode
Figure 3 describes the set-up used to transfer under equi-pressure mode. It is composed of three main parts: the PMHP 600-600, the 500 mL-HPB containing seawater sample (entitled thereafter HPB 1) and the HPB with a labeled tracer to measure activity or HPB with a culture medium (entitled thereafter HPB 2). To facilitate handling and to reduce experiment costs, 50 mL HPBs (2730-4000, Top Industrie SA) may be used instead of 500 mL HPBs. Both volumes of HPB can be used to perform a transfer in equi-pressure mode if the HPBs are equipped with a floating-piston inside.
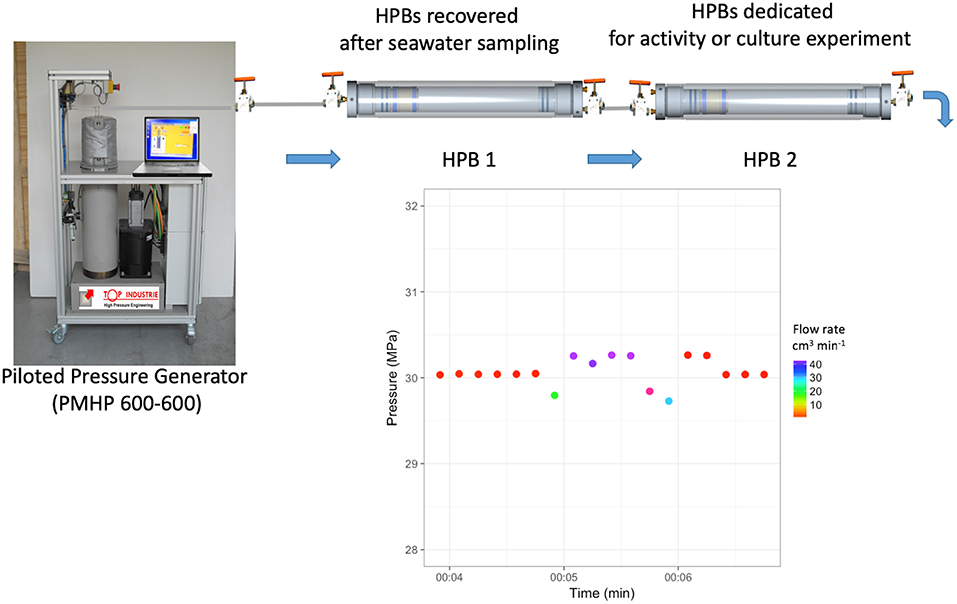
Figure 3 . Transfer in equi-pressure mode. After sampling HPBs dedicated to sample (HPB 1) are connected at bottom end-cap to piloted pressure generator (PPG) (PMHP 600-600) and at top end-cap to HPB dedicated to activity measurement or culture experiments (HPB 2), previously pressurized at the working pressure. In the example, the PMHP 600-600 and the HPB2 are preconditioned to 30 MPa, the same hydrostatic pressure of the 3,000 m-depth sample of the HPB1. During the transfer, the PMHP 600-600 prevents pressure loss by adding milliQ water at the working pressure into the lower part of HPB 1, the sample being isolated by the floating-piston. The equivalent volume of the seawater sample enters HPB 2, which is managed by a handle valve at the bottom end-cap. Data is recorded during transfer in equi-pressure mode with a time-step of 10 s. The transfer takes about 2 min for a volume of 40 mL with an accuracy of <0.5 MPa.
Firstly, HPB 2 is filled with the labeled tracer or the culture medium and pre-conditioned at the in situ temperature. This operation is done at atmospheric pressure using a precision syringe in sterile conditions. Then, the HPB 2 is pressurized at the same pressure as the HPB 1 to equilibrate the pressure between both HPBs. Finally, both hand valves of the HPBs (at the top) are connected using a sterile 1/8″ (3.2 mm O.D.) stainless tube. The bottom part of the HPB 1 is connected to the PMHP 600-600. The top hand-valve of HPB 1 is opened to equilibrate the pressure with the small stainless-tube connection. The top hand-valve of HPB2 can be opened. The bottom part of the HPB 2, also fitted with a 1/8″ hand valve, is then slowly opened by the operator to create a weak leak, the PMHP 600-600 compensates the pressure loss by an increase in the flow rate. The PMHP 600-600 regulates the hydrostatic pressure by injecting distilled water into the bottom part of the HPB1, separated from the original deep-sea sample by the floating-piston. The operator regulates the volume using the end hand-valve. The PMHP 600-600 regulates the hydrostatic pressure in both HPBs, while the operator manages the transferred volume. Hence, the original deep-sea sample is transferred from HPB 1 into HPB 2 at constant pressure. HPBs are covered with a survival blanket to limit temperature change. From 500 mL samples, it is possible to transfer sub-samples of different volumes to perform replicates for metabolic activities or culture experiments under in situ pressure conditions. Less than two minutes is required to transfer 40 mL of sub-sample with a pressure accuracy of < 0.5 MPa (see the graph in Figure 3 and animated diagram in Supplementary Video 2 ).
Example of Field Application
In situ sampling and incubation experiment under high-hydrostatic pressure.
The experiment was conducted during the PEACETIME cruise ( http://peacetime-project.org/ ) in May 2017 in the Ionian Sea. Seawater was sampled from 3,000 m-depth (Station ION, 35.49N, 19.78E) using two HPSUs, one under in situ conditions (30 MPa) and another one decompressed during up-cast (HPSU mounted without check valve) and incubated at atmospheric pressure (0.1 MPa). Details of the PEACETIME cruise will be fully described in a special issue soon. Incubations were carried out at in situ temperature (i.e., at 13°C in the deep Mediterranean Sea) for 7 days. At the end of this first incubation (I 1 in Figure 4 ), fifty milliliters (50 mL) were transferred in equi-pressure mode into another HPB previously filled with 450 mL 0.2-μm-pore size filtered-seawater (to remove particulate matter and microorganisms), obtained at the same time and at the same depth using regular Niskin bottles for the second incubation (I 2 ). The remaining volume was filtered, in <15 min, through 0.2-μm-pore-size filters (Millipore ® , GPWP04700) without any fixation and stored at −80°C for DNA/RNA metabarcoding analysis. A third incubation (I 3 ) is performed with the same protocol. A total of three incubations were performed maintaining in situ pressure (HP) or decompressed and incubated at atmospheric pressure conditions (DEC) (see workflow in Figure 4 ). The concentration of dissolved-oxygen was monitored during the incubation using optode sensors (see section In situ oxygen consumption and Figure 4 ).
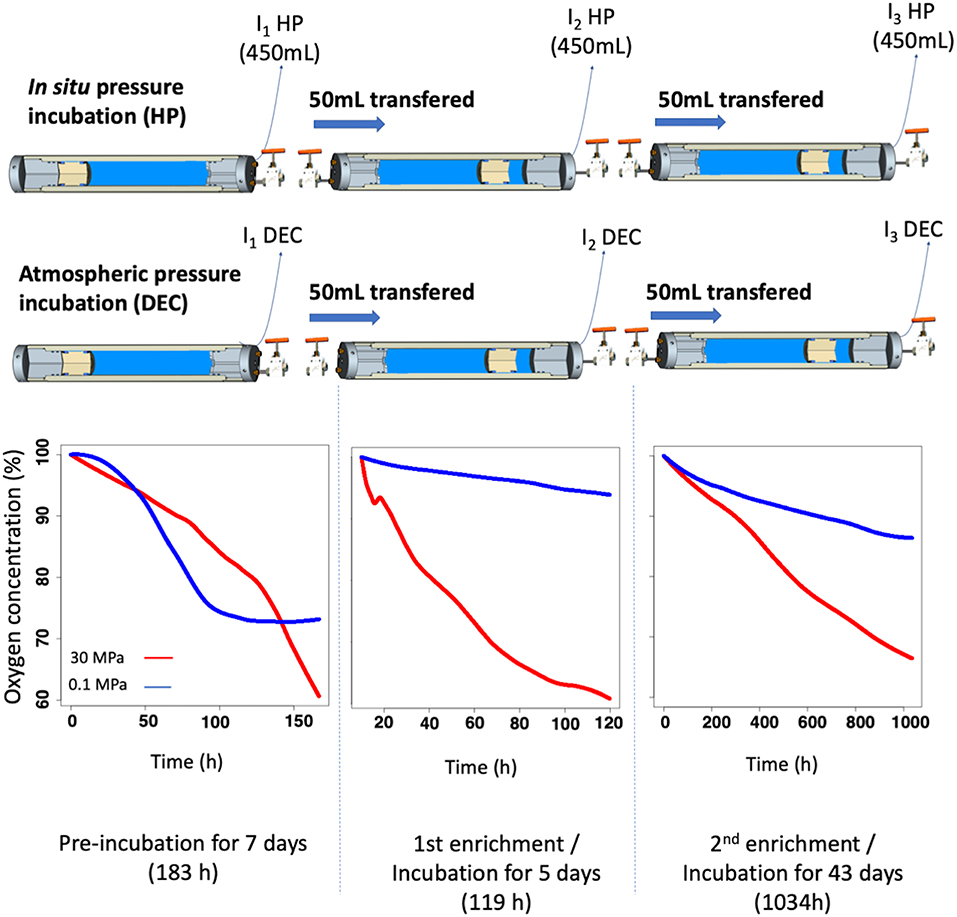
Figure 4 . Timeline of the incubation experiment. Deep-sea samples were recovered at 3,000 m-depth and incubated at in situ pressure conditions (HP) or after decompression at atmospheric pressure conditions (DEC). Samples were firstly incubated at in situ temperature over 7 days. Then, the second incubation was made by transferring, in equi-pressure mode for each condition, 50 mL of the first incubated sample into a new high-pressure bottle (HPB) containing 450 mL of 0.2 μm-filtrated and sterilized seawater, sampled at the same depth. The remaining 450 mL of the first-incubated HPBs were then quickly sampled and conditioned for sequencing analysis (T 0 HP and T 0 DEC). At the end of the 2nd incubation, a third incubation was made according to the same protocol. At the end of each incubation the remaining 450 mL was conditioned for sequencing analysis and named T 1 HP/T 1 DEC and T 2 HP/ T 2 DEC, respectively. Dissolved-oxygen concentration was used to determine the incubation time. Graph plots represent dissolved-oxygen concentration (expressed in percent of initial dissolved-oxygen concentration) against time (h).
Prokaryotic Heterotrophic Production
Prokaryotic heterotrophic production (PHP) was measured by incorporating L-[4,5- 3 H]-Leucine, ( 3 H-Leu, 109 Ci mmol −1 of specific activity, PerkinElmer ® ) to get a final concentration of 10 nM in HPBs. Saturation concentrations were previously defined by multi-concentration kinetic experiments at atmospheric pressure (data not shown) and according to previous experiments (e.g., Tamburini et al., 2002 ). Transfer and sub-sampling of high-pressure samples were performed using a piloted pressure generator to ensure that hydrostatic pressure was maintained throughout the procedure within the mobile MIO-HPLab container (certified for the use of radiolabeled compounds). Figure 3 and Supplementary Video 2 present an example of the transfer from the HPB containing the 3,000 m-samples, obtained with the HPSU (HPB1), and the HPB pre-filled with the 3 H-Leu (HPB2). In this case, forty millimeters of deep-sea samples were transferred into 3 independent HPBs (triplicate), amended with 3 H-Leu, for samples maintained at in situ pressure conditions (HP). In parallel, triplicate 40 mL formaldehyde-killed blanks and triplicate 40 mL decompressed atmospheric pressure (DEC) samples were incubated with a final concentration of 10 nM of 3 H-Leu. All the replicates (3 HP, 3 DEC, 3 blanks) were incubated at in situ temperature (13°C) in a Peltier-cooled incubator (Memmert ® IPP750 plus). After 10 h of incubation, samples were fixed with 2% final concentration formaldehyde and stored at 4°C until filtration. The following protocol is detailed in Kirchamn (1993) . To calculate the PHP, we used the empirical conversion factor of 1.55 ng C pmol −1 of incorporated 3 H-Leu according to Simon and Azam (1989) assuming that isotope dilution was negligible under these saturating concentrations. The concentration of 3 H-Leu within HPBs was checked at the end of the experiment based on 100 μL of samples.
In situ Oxygen Consumption
To measure, dissolved-oxygen consumption inside HPBs, we modified the top end-cap of the HPB with a sapphire window for optical measurement (see details in “High-pressure bottles” section and Supplementary Image 2 ). We have adapted an available solution based on an optical method using a non-invasive planar optode method which provides high-frequency measurements. The optical oxygen-sensor spot was glued with silicon glue onto the sapphire window directly inside the HPB rather than using a flow-through cell as in the pressure incubation system of McNichol et al. (2016) . The oxygen sensor, containing the photoluminescent quenching dye, is produced by Presens GmbH ® (Pst3, detection limit 15 ppb, ≈ 0.47 μM) or by PyroScience GmbH ® (OXSP5, detection limit 0.3 μM). On the other side of the sapphire window, a polymeric optic-fiber was held against the sapphire window and was connected to a data logger. We used OXY-10 mini device for Presens GmbH ® or FireStingO2 (fiber-optic oxygen meter) coupled to TeX4 (temperature extension module) for PyroScience GmbH ® . Both data loggers shone a beam of light at a precise wavelength (for Presens 485 nm/620 nm, for PyroScience 620 nm/760 nm) onto the optode spot from the outside. Oxygen concentration data were then collected every minute during the incubation. Particular attention must be given to maintaining a constant temperature (here the samples were maintained at 13 ± 0.1°C) during the whole incubation to avoid a temperature sensor dependence signal during the experiment. The optodes were calibrated manually using a two-point calibration procedure. All optodes are intercalibrated individually and intercompared. Hydrostatic pressure, temperature and salinity are compensated for using algorithms proposed by McNeil and D'Asaro (2014) and García et al. (1992) . In the Figure 4 , dissolved-oxygen concentration is expressed as a percentage of initial concentration to normalize the differences in absolute concentration at the initial time of each incubation.
DNA and RNA Extraction and PCR Amplification
The 16S-rDNA (revealing all taxa present in a given sample) and 16S-rRNA (revealing taxa actively transcribing RNA) were taken from the same filter. 16S-rDNA and 16S-rRNA were extracted on two different pieces of filter. Each piece of filter was treated with TE-Lysis buffer (20 mM Tris, 25 mM EDTA, 1 μg μL −1 Lysozyme) followed by 10% SDS. The extractions were performed twice with an equal volume of phenol:chloroform:isoamyl alcohol pH8 16S-rDNA and pH 6 16S-rRNA. Then 16S-rRNA samples were treated with TurboDNase TM (Ambion ® , Thermo Fisher Scientific Corp.) and reverse transcribed into cDNA by RT-PCR using SuperScript ® IV Reverse Transcriptase with random primers (Life Technologies, Thermo Fisher Scientific Corp.). For ribosomal diversity analysis, the V4 region of the bacterial and archaeal 16S rDNA genes and 16S cDNA product were amplified using universal primer sets ( Caporaso et al., 2012 ), 515F-Y (5′ -GTGYCAGCMGCCGCGGTAA-3′, Parada et al., 2016 ) and 806RB (5′-GGACTACNVGGGTWTCTAAT-3′, Apprill et al., 2015 ) and using 2.5 U/50 μL TaKaRa PrimeSTAR ® GXL DNA polymerase (OZYME). The 16S amplicons were sequenced by the MiSeq Illumina (paired end 2* 250) platform GeT of Genotoul ( https://get.genotoul.fr/en/ ). 16S-rDNA and 16S-rRNA raw reads sequences are deposited on public database GeneBank (accession number are ranged between SRR8503024 to SRR8503035).
16S rDNA/rRNA Sequences Analysis
The paired-end raw reads were firstly overlapped and merged by platform GeT of Genotoul. The analysis of the 16S-assembled data relied on the use of QIIME 1.91 ( Kuczynski et al., 2012 ). The removal of low quality bases and chimera sequences were performed by QIIME 1.91 script and UCHIME ( Edgar et al., 2011 ), respectively. The high-quality sequences were then clustered into the Operational Taxonomic Unit (OTU) using the UCLUST algorithm and a threshold of 97% of sequence identity. The taxonomic assignment of representative sequences of OTUs was performed using the SILVA123 database ( Edgar, 2010 ; Quast et al., 2013 ). The OTU table was filtered for low abundance OTUs ( Bokulich et al., 2013 ). Finally, sub-sampling normalization, alpha and beta diversity were characterized by Phyloseq, an open-source software package project for R ( www.r-project.org ) ( R Core Team, 2008 ; McMurdie and Holmes, 2013 ).
Results and Discussion
In this article, we describe a field application using the pressure-retaining sampler and high-pressure systems that we developed. Samples taken from 3,000 m-depth were obtained in the Mediterranean Sea during the multidisciplinary cruise PEACETIME in May 2017. Three HPSUs were implemented on the CTD-carousel used during the PEACETIME cruise, one HPSU dedicated to the measurement of PHP and the other two for the incubation experiment described in Figure 4 .
Prokaryotic Activity Measurements
The prokaryotic heterotrophic production (PHP) measurements were performed to characterize the prokaryotic community lifestyle status with respect to hydrostatic pressure ( Tamburini et al., 2013 ). PHP was 3.2-fold higher for samples maintained at in situ (PHP HP = 0.93 ± 0.13 ng C L −1 , n = 3) than those decompressed and incubated at atmospheric pressure conditions (PHP DEC = 0.29 ± 0.01 ng C L −1 , n = 3). Thus, the natural 3,000 m-depth prokaryotic assemblage sampled can be defined globally as piezophilic (that means adapted to in situ high pressure conditions) according to Tamburini et al. (2013) and Wannicke et al. (2015) . This global adaptation of the prokaryotic assemblage to pressure is supported by the prokaryotic respiration rate (PR) measurements derived from oxygen consumption rates within the two HPBs during the incubation experiment. One set of samples was maintained at in situ hydrostatic pressure (HP) and the other was decompressed and incubated at atmospheric pressure (DEC). Table 1 and plots presented in Figure 4 summarize the O 2 -consumption results. Oxygen consumption is clearly always higher in HP than in DEC conditions as exemplified by the third incubation experiment where only 14% of the O 2 is consumed in DEC conditions compared to 34% in HP conditions after 43 days of incubation (see O 2 graph-plots in Figure 4 ). Results in Table 1 show that for each incubation experiment, PR was between 1.6 and 7.0-folds higher under in situ pressure conditions than in incubations carried out after decompression and incubated in atmospheric conditions. Furthermore, the sampling strategy of the incubation experiments was adjusted using real-time monitoring of oxygen concentrations. All incubation experiments were performed until oxygen consumption reached a maximum of 40%.
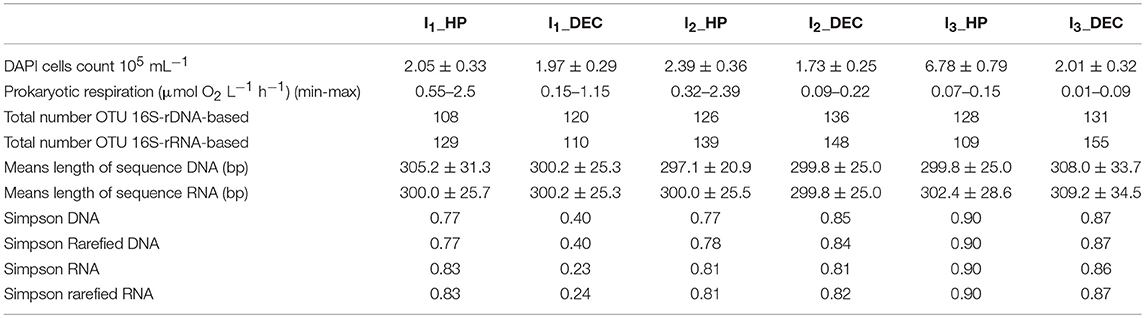
Table 1 . Total cells count (stained with DAPI), range of prokaryotic respiration, number of observed operational taxonomic units (OTUs), 16S-rDNA- and 16S-rRNA-based sequence lengths, and alpha-diversity indexes for enrichment incubation experiments ( Figure 4 ).
Prokaryotic Diversity and Community Structure (16S rDNA and rRNA)
The prokaryotic diversity and community structure shifts were characterized by 16S rDNA (the resident community) and 16S rRNA (the active community) gene sequencing according to experimental conditions (HP and DEC). Beyond RNA instability and bias originating from DNA/RNA extraction and amplification, the rRNA- overcomes rDNA-based approach for detecting live prokaryotic cells in water ( Li et al., 2017 ).
The amplicon sequencing followed by trimming and normalization processes generated 19420 16S rDNA and 16S rRNA high quality reads (average length 302 bp) per sample ( Table 1 ). In addition, the number of observed OTUs ranged from 108 to 155 over time with no significant difference between rDNA and rRNA analysis. The decrease of the specific richness during the experiment was indicated by the Simpson index, which increases from 0.77 to 0.90 and from 0.40 to 0.87 within HP and DEC samples, respectively. Moreover, the comparison of the OTUs relative abundances shared between rDNA and rRNA [log(rDNA/rRNA)] showed a distribution around the 1:1 bar, especially for the more abundant ones ( Supplementary Image 4 ). Beyond the pitfalls linked to the overestimation or underestimation of OTUs abundances, this result suggests that the active community corresponded to almost the entire resident community. This finding is fully supported by a non-metric multidimensional scaling (NMDS) analysis that highlighted close positions for rDNA and rRNA samples with common experimental conditions ( Figure 5 ). In contrast, the shifts observed in prokaryotic community structure over time are driven by the different experimental conditions, confirming a previous study ( Wannicke et al., 2015 ). Indeed, HP vs. DEC incubation conditions were mainly discriminated by the absence of Colwelliacea and Bacteriovracaceae in DEC incubations (NMDS1 axis). This is already the case at the end of the 1st incubation (I 1 _HP vs. I 1 _DEC) and reinforces the results that decompression impacts not only prokaryotic activity as shown in this study and elsewhere ( Tamburini et al., 2013 ; Wannicke et al., 2015 ) but also affects the community composition ( La Cono et al., 2009 , 2015 ) or the gene expression ( Edgcomb et al., 2016 ).
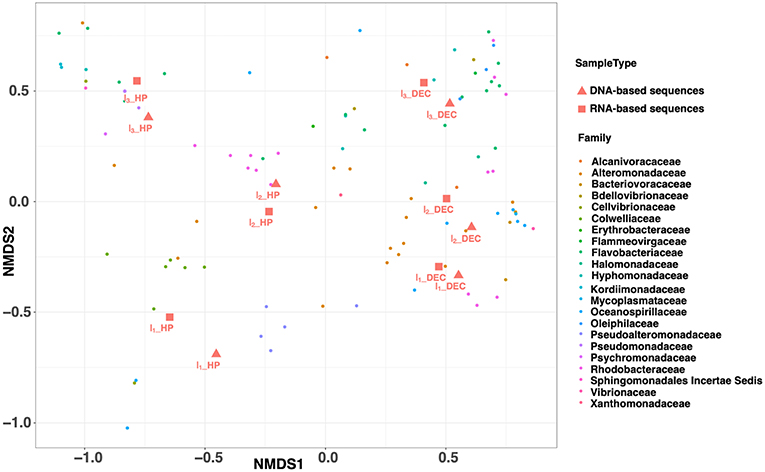
Figure 5 . NMDS ordination plot (Bray–Curtis distance matrix) from incubation experiment performed with deep-seawater sample maintained at in situ condition (HP) and after decompression and incubated at atmospheric pressure (DEC). This graph shows two clusters: on one side the HP samples and on the other the DEC samples. Prefix D and R before the name for the sample is for DNA- or RNA-based sequences, respectively. The NMDS stress is equal to 0.03.
For each experimental condition (HP and DEC), variation in community structure over time (incubation time-series) was observed and driven by the NMDS2 axis ( Figure 5 ). This suggests that sufficient carbon and energy is available in the deep-sea water sampled at 3,000 m-depth used as the “medium” in our incubation experiments. Figure 6 shows the shifts in prokaryotic community structure from the ten most abundant families (corresponding to four classes) during the course of the incubation experiments. After the 1 st incubation experiment (I 1 ) no Archaea were detected in either condition whilst they can reach 50% of the prokaryotic community in the deep ocean ( Karner et al., 2001 ; Herndl et al., 2005 ; Tamburini et al., 2009a ). The absence of Archaea genes sequenced in the incubation experiment is confirmed by the RT-qPCR quantification showing more than 4-orders of magnitude between the number of Archaea and Bacteria gene copies per milliliter (data not shown). In DEC conditions (I 1 _DEC), the class Gammaproteobacteria accounted for 93% of the total sequences and was almost exclusively represented by the family Alteromonadaceae ( Figure 6 ). Conversely, in HP conditions (I 1 _HP), the prokaryotic community was also dominated by the class Gammaproteobacteria (99% of the total sequences) but included various families such as Alteromonadaceae, Oceanospirillaceae, Pseudoalteromonas , and Colwelliaceae . The family Alteromonadaceae accounted only for 15% (of both 16S rDNA- and rRNA sequences), while Colwelliaceae dominated with 53 and 25% of 16S rDNA- and rRNA sequences, respectively. In our study, the family Colwelliaceae was mainly made up of the genus Colwellia (more than 50%) which contained isolates described as piezophilic ( Deming et al., 1988 ; Nogi et al., 2004 ; Eloe et al., 2011 ). The proportions of family Pseudoaltermonadaceae reached 22 and 25% of 16S rDNA- and rRNA sequences, respectively. Interestingly, Oceanospirillaceae represented 9% of 16S rDNA sequences but 33% of 16S rRNA sequences at I 1 _HP. The family Oceanospirillaceae is one of the rare families containing piezophilic isolates ( Cao et al., 2014 ).
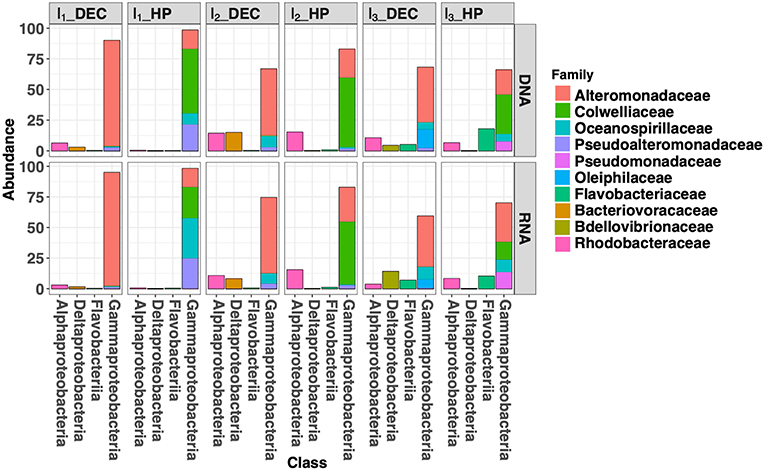
Figure 6 . Bar plot of the 10 most abundant families into the four main classes during incubation experiments of deep-seawater samples maintained at in situ pressure conditions (HP) and after decompression and incubated at atmospheric pressure conditions (DEC).
At the end of the third incubation experiment (I 3 , Figure 4 ), the active community (rRNA) of the Gammaproteobacteria decreased around 60 and 70% in DEC and HP conditions, respectively. Under DEC conditions, this decrease was in favor of Bdellovibrionaceae (family Deltaproteobacteria ) which is mainly represented by the genus OM27, previously described as bacterial predatory ( Fuchs et al., 2005 ; Orsi et al., 2016 ) and was only found in the DEC condition. This result suggests that high hydrostatic pressure could initiate growth repression of these microorganisms within this family. In contrast, at I 3 _HP, an increase in the abundance of families Rhodobacteraceae ( Alphaproteobacteria class) and Flavobacteriaceae ( Flavobateria class) was observed and reached 10 and 8% of the rRNA total sequences, respectively. Both of these families were absent from the “active community” at I 1 _HP. The family Rhodobacteraceae was found in both DEC and HP conditions in proportions ranging from 6 to 15% and are ubiquitous from the surface to the deep ocean ( Moran et al., 2003 ). Members of the family Flavobacteriaceae have shown the ability to degrade high molecular dissolved organic matter (HMW-DOM) such as chitin, agar or particulate organic matter (POM) that characterize the deep-sea environment ( Kirchman, 2002 ; Fernández-Gómez et al., 2013 ). It is widely known that the deep-sea waters are depleted in carbon and energy sources. Consequently, deep-sea microorganisms harbored unique metabolic capabilities with respect to the degradation of complex organic matter and were supported by genomic and transcriptomic analysis ( Vezzi et al., 2005 ; Delong et al., 2006 ) as well as the measurements of degradation of refractory organic matter in deep water compared with surface water ( Hoppe and Ullrich, 1999 ; Teira et al., 2006 ; Tamburini et al., 2009a ; Boutrif et al., 2011 ).
Conclusion and Perspectives
Conserving the in situ conditions when sampling the deep ocean is becoming a major concern for the scientific community and many have tried to find solutions to sample without decompressing the samples. For example, Shillito and collaborators developed PERISCOP to sample deep-sea macro-organisms (e.g., shrimps, crabs), under in situ conditions without decompression issues. This was then adapted by BALIST into IPOCAMP for use in physiology and behavioral experiments ( Shillito et al., 2008 , 2014 ; Ravaux et al., 2013 ). Likewise, McNichol et al. (2016) developed a specific device to sample hydrothermal vent fluids under in situ conditions and to study microbial metabolism associated with fluid biogeochemistry. Peoples et al. (2019) proposed a pressure-retaining sampler (coupled to a Lander) capable of collecting hadal seawaters under in situ conditions during recovery. Finally, Parkes et al. (2009) have proposed high-pressure sampler systems to study prokaryotic subseafloor sediments.
Based on the prototype proposed by Bianchi et al. (1999) and our experience with more than 200 deep-water samples collected under in situ pressure conditions, we propose a 6,000 m-seawater pressure-retaining sampler ready-to-use for the scientific community. The various improvements implemented promote the success of high-pressure sampling, sub-sampling, and transfer of samples in equi-pressure mode in replicate. In addition, it is also possible to directly measure oxygen consumption at high hydrostatic pressure and to use radiolabeled compounds in accordance with health and safety standards. Such methodology can be an outstanding tool in isolating new piezophilic strains without any pressure losses during the experiment.
Apart from collecting samples and maintaining them under in situ conditions, it is important to note that this system and specifically HPBs are versatile. For example, 316L stainless-steel with PEEK coating HPBs can be selected for cultivation under anoxic conditions (e.g., sulfato-reducing bacteria) they don't corrode. HPBs and PPGs can be used to perform laboratory experiments such as for sinking particle simulation experiments ( Tamburini et al., 2006 , 2009b ; Riou et al., 2017 ). To our knowledge, several teams already have the same pressure-retaining sampler (Deep Carbon Observatory, DCO—Sloan Foundation; Hadal Science and Technology Research Center, HAST, Shangai, China; Sanya Institute of Deep Sea Science and Engineering, SIDSSE, China Academy of Science). Using the same HPBs, IBIS is being developed by IFREMER for the sampling of hydrothermal fluids.
The incubation experiment, presented in this study, is an example of a field application using the pressure-retaining sampler and associated high-pressure systems. This illustrates its fully operational use during our field experiment dedicated to deep-sea microbial oceanography. Using prokaryotic diversity and community structure based on 16S rDNA- and rRNA-sequences, we have shown that the diversity decreased in both HP and DEC conditions and that the community structure evolved differently according the pressure conditions. Further studies will be conducted to reveal the metabolic pathways and microbial taxa involved in the biogeochemical transformation of the organic matter in the dark ocean, as many important ecological and biogeochemical processes, linked to the biological carbon pump, take place in this the largest habitat of the biosphere.
Author Contributions
MG and CT wrote the initial draft and coordinated the drafting of the paper, and participated in the experimental design. The high-pressure experiments were carried out by MG, CT, MR, and NB. SG and PB participated in molecular biological work. MG and FA performed sequencing analyses. All authors contributed to the discussion and the writing of the paper.
The project leading to this publication has received funding from European FEDER Fund under project 1166-39417. It is a contribution to the Mediterranean Institute of Oceanography AT-Pompe Biologique, and to the Labex OT-Med (ANR-11-LABEX-0061, www.otmed.fr ) funded by the Investissements d'Avenir, French Government project of the ANR, through the AMidex project (ANR-11-IDEX-0001-02). It is also a contribution to the PEACETIME project ( http://peacetime-project.org ), a joint initiative of the MERMEX and ChArMEx components supported by CNRS-INSU, IFREMER, CEA, and Météo-France as part of the program MISTRALS coordinated by INSU.
Conflict of Interest Statement
The authors declare that the research was conducted in the absence of any commercial or financial relationships that could be construed as a potential conflict of interest.
Acknowledgments
Authors would like to acknowledge PEACETIME project (MISTRALS CNRS INSU, doi: 10.17600/17000300) for opportunity to participate to the cruise. We would also like to acknowledge Top Industrie SAS ( https://www.top-industrie.com ) for all schematic drawing, and C. Gojak and K. Mahiouz from DT-INSU CNRS for mechanical improvement. Authors also thank D. Lefevre for discussions on oxygen measurements using oxygen planar optodes within high-pressure bottles. We thank F. Le Moigne for discussions and T. L. Bentley for English improvement. We thank the captains and crews of RV Pourquoi Pas? for cruise assistance and C. Guieu and K. Desboeufs, Chief scientists of PEACETIME.
Supplementary Material
The Supplementary Material for this article can be found online at: https://www.frontiersin.org/articles/10.3389/fmicb.2019.00453/full#supplementary-material
Supplementary Image 1 . Top end cap with optic measurement. (1) Optic fiber, (2) Temperature sensor (pt100), (3) Inlet/outlet 1/8″ diameter (3.2 mm O.D.), (4) O'ring, (5) Planar optode (diameter 5 mm), (6) Sapphire window, (7) Titanium screw. This modified top end-cap can be fitted indifferently on TA6V HPBs or on 316L stainless-steel with PEEK coating HPBs. However, the latter has to be pre-conditioned to equilibrate O 2 concentration with PEEK coating. In this experiment, we have used TA6V HPBs to follow the oxygen concentration during the experiments both at atmospheric and in situ pressure conditions.
Supplementary Image 2 . Schematic drawing of sampling valve fitted on High-Pressure Sampler Unit (HSU). 1. Nylon wire; 2. Stainless-steel trigger; 3. Stainless-steel frame for valve; 4. Modified swagelock valve (réf: SS-84PS4); 5. Modified handle to link trigger at spring; 6. sample inlet, 7. spring. In this view sampling valve is set in close position before sampling (A) . At the sampling depth nylon wire is disconnected to carrousel trigger, modified Swagelock ® valve move to opening position performing a quarter of a rotation by power of the extended spring (B) .
Supplementary Image 3 . Photograph showing different point of view of the mobile MIO-HPLab container. This mobile laboratory has been constructed in a 20 feet container. It is composed of two piloted pressure genrators (PPGs), four temperature regulated water baths with two temperature coolers dedicated for High-Pressure Sampler Unit (HPSU) and particle sinking experiments (PASS) experiments ( Tamburini et al., 2009b ), and a reinforced Peltier-cooled incubator Memmert IPP 750 oven for HPBs incubation. The mobile laboratory (MIO-HPLab container) is also certified to use radiolabeled during oceanographic cruises.
Supplementary Image 4 . RNA:DNA ratios from RNA-based and DNA-based relative log transformed abundance of OTU. This ratio enables us to estimate a proportion of active prokaryote for each sample. The black line is 1:1 bar. OTU are distributed around the line which indicates that the microbial communities identified in the sample are also active.
Supplementary Video 1 . Animated schematic drawing in transparency of the front and back views of the High-Pressure Sample Unit (HPSU). (A) During the descent of the CTD-carousel. The inlet-valve is in close position; (B) During the sampling at the chosen depth after firing the CTD-Carousel and the inlet-valve is in open position. The numbers are the same than in Figure 1: 7. exhaust tank; 8. Polypropylene main frame; 9. Push rod for attachment to a rosette system. When the (10) inlet-valve is opened by magnetically activated lanyard release, the seawater enters the hydraulic circuit through a 1/8″ (3.2 mm O.D.) stainless-steel tube (11), via one check valve (12), to fill in the HPB (13), the pressure accumulator (14) and the aero-hydraulic pressure sensor (15).
Supplementary Video 2 . An animated diagram showing a transfer in equi-pressure mode between two High-Pressure Bottles (HPBs). HPB1 is the high-pressure bottle containing the 3,000 m-depth sample maintained under in situ pressure conditions. HPB2 is the high-pressure bottle containing radiolabeled compounds, such as, 3 H-Leucine to measure prokaryotic heterotrophic production (PHP). Forty milliliters of 3000 m-depth seawater sample from HPB1 are transferred into HPB2 containing 10 nM of aqueous 3 H-Leucine. This process is performed, securely, in replicate inside the mobile MIO-HPLab container.
Apprill, A., McNally, S., Parsons, R., and Weber, L. (2015). Minor revision to V4 region SSU rRNA 806R gene primer greatly increases detection of SAR11 bacterioplankton. Aquat. Microb. Ecol . 75, 129–137. doi: 10.3354/ame01753
CrossRef Full Text | Google Scholar
Aristegui, J., Gasol, J. M. M., Duarte, C. M. M., and Herndl, G. J. (2009). Microbial oceanography of the dark ocean's pelagic realm. Limnol. Ocean 54, 1501–1529. doi: 10.4319/lo.2009.54.5.1501
Bartlett, D. H., Lauro, F. M., and Eloe, E. A. (2007). “Microbial adaptation to high pressure,” in Physiology and Biochemistry of Extremophiles , eds C. Gerday and N. Glansdorff (Washington, DC: American Society of Microbiology), 333–348. doi: 10.1128/9781555815813.ch25
Bianchi, A., Garcin, J., and Tholosan, O. (1999). A High-pressure serial sampler to measure microbial actvity in the deep sea. Deep Sea Res. Part I 46, 2129–2142. doi: 10.1016/S0967-0637(99)00039-4
Bokulich, N. A., Subramanian, S., Faith, J. J., Gevers, D., Gordon, J. I., Knight, R., et al. (2013). Quality-filtering vastly improves diversity estimates from Illumina amplicon sequencing. Nat. Methods 10, 57–59. doi: 10.1038/nmeth.2276
PubMed Abstract | CrossRef Full Text | Google Scholar
Boutrif, M., Garel, M., Cottrell, M. T., and Tamburini, C. (2011). Assimilation of marine extracellular polymeric substances by deep-sea prokaryotes in the NW Mediterranean Sea. Environ. Microbiol. Rep . 3, 705–709. doi: 10.1111/j.1758-2229.2011.00285.x
Cao, Y., Chastain, R. A., Eloe, E. A., Nogi, Y., Kato, C., and Bartlett, D. H. (2014). Novel psychropiezophilic oceanospirillales species Profundimonas piezophila gen. nov., sp. nov., isolated from the deep-sea environment of the Puerto Rico trench. Appl. Environ. Microbiol . 80, 54–60. doi: 10.1128/AEM.02288-13
Caporaso, J. G., Lauber, C. L., Walters, W. A., Berg-Lyons, D., Huntley, J., Fierer, N., et al. (2012). Ultra-high-throughput microbial community analysis on the Illumina HiSeq and MiSeq platforms. ISME J . 6, 1621–1624. doi: 10.1038/ismej.2012.8
Cho, B. C., and Azam, F. (1988). Major role of bacteria in biogeochemical fluxes in the ocean's interior. Nature 332, 441–443.
Google Scholar
Delong, E. E. F., Preston, C. C. M., Mincer, T., Rich, V., Hallam, S. J., Frigaard, N., et al. (2006). Community genomics among stratified microbial assemblages in the ocean's interior. Science 311, 496–503. doi: 10.1126/science.1120250
Deming, J. W., Somers, L. K., Straube, W. L., Swartz, D. G., and Macdonell, M. T. (1988). Isolation of an obligately barophilic bacterium and description of a new genus, colwellia gen. nov. Syst. Appl. Microbiol . 10, 152–160. doi: 10.1016/S0723-2020(88)80030-4
Edgar, R. C. (2010). Search and clustering orders of magnitude faster than BLAST. Bioinformatics 26, 2460–2461. doi: 10.1093/bioinformatics/btq461
Edgar, R. C., Haas, B. J., Clemente, J. C., Quince, C., and Knight, R. (2011). UCHIME improves sensitivity and speed of chimera detection. Bioinformatics 27, 2194–2200. doi: 10.1093/bioinformatics/btr381
Edgcomb, V. P., Taylor, C., Pachiadaki, M. G., Honjo, S., Engstrom, I., and Yakimov, M. (2016). Comparison of Niskin vs. in situ approaches for analysis of gene expression in deep Mediterranean Sea water samples. Deep Sea Res. Part II 129, 213–222. doi: 10.1016/j.dsr2.2014.10.020
Eloe, E. A., Shulse, C. N., Fadrosh, D. W., Williamson, S. J., Allen, E. E., and Bartlett, D. H. (2011). Compositional differences in particle-associated and free-living microbial assemblages from an extreme deep-ocean environment. Environ. Microbiol. Rep . 3, 449–458. doi: 10.1111/j.1758-2229.2010.00223.x
Fernández-Gómez, B., Richter, M., Schüler, M., Pinhassi, J., Acinas, S. G., González, J. M., et al. (2013). Ecology of marine bacteroidetes: a comparative genomics approach. ISME J . 7, 1026–1037. doi: 10.1038/ismej.2012.169
Fuchs, B., Woebken, D., Zubkov, M., Burkill, P., and Amann, R. (2005). Molecular identification of picoplankton populations in contrasting waters of the Arabian Sea. Aquat. Microb. Ecol . 39, 145–157. doi: 10.3354/ame039145
García, H. E., Gordon, L. I., and Millero, F. (1992). Oxygen solubility in seawater: Better fitting equations. Limnol. Oceanogr . 37, 1307–1312. doi: 10.4319/lo.1992.37.6.1307
Herndl, G. J., Reinthaler, T., Teira, E., Van Aken, H., Veth, C., Pernthaler, A., et al. (2005). Contribution of Archaea to total prokaryotic production in the deep Atlantic Ocean. Appl. Environ. Microbiol. 71, 2303–2309. doi: 10.1128/AEM.71.5.2303
Hoppe, H., and Ullrich, S. (1999). Profiles of ectoenzymes in the Indian Ocean: phenomena of phosphatase activity in the mesopelagic zone. Aquat. Microb. Ecol . 19, 139–148. doi: 10.3354/ame019139
Jannasch, H. W., Wirsen, C. O., and Winget, C. L. (1973). A bacteriological pressure-retaining deep-sea sampler and culture vessel. Deep. Res. Oceanogr . 20, 661–664. doi: 10.1016/0011-7471(73)90033-8
Karner, M. B., Delong, E. F., and Karl, D. M. (2001). Archaeal dominance in the mesopelagic zone of the Pacific Ocean. Nature 409, 507–510. doi: 10.1038/35054051
Kim, S. J., and Kato, C. (2010). “Sampling, isolation, cultivation, and characterization of piezophilic microbes,” in Handbook of Hydrocarbon and Lipid Microbiology , ed K. N. Timmis (Berlin; Heidelberg: Springer), 3869–3881.
Kirchamn, D. L. (1993). “Leucine incorporation as a measure of biomass production by heterotrophic bacteria,” in Handbooks of Methods in Aquatic Microbial Ecology , eds P. F. Kemp, B. F. Sherr, E. B. Sherr, J. J. Cole (Boca Raton, FL: Lewis Publishers), 509–5012.
Kirchman, D. L. (2002). The ecology of cytophaga-flavobacteria in aquatic environments. FEMS Microbiol. Ecol . 39, 91–100. doi: 10.1016/S0168-6496(01)00206-9
Kuczynski, J., Stombaugh, J., Walters, W., González, A., Caporaso, J. G., and Knight, R. (2012). Using QIIME to Analyze 16S rRNA gene sequences from nMicrobial communities. Curr. Protoc. Microbiol. Chapter 10, Unit 10.17. doi: 10.1002/0471250953.bi1007s36
La Cono, V., Smedile, F., La Spada, G., Arcadi, E., Genovese, M., Ruggeri, G., et al. (2015). Shifts in the meso- and bathypelagic archaea communities composition during recovery and short-term handling of decompressed deep-sea samples. Environ. Microbiol. Rep . 7, 450–459. doi: 10.1111/1758-2229.12272
La Cono, V., Tamburini, C., Genovese, L., La Spada, G., Denaro, R., and Yakimov, M. M. (2009). Cultivation-independent assessment of the bathypelagic archaeal diversity of Tyrrhenian Sea: Comparative study of rDNA and rRNA-derived libraries and influence of sample decompression. Deep Sea Res. Part II Top. Stud. Oceanogr. 56, 768–773. doi: 10.1016/j.dsr2.2008.07.025
Lauro, F. M., and Bartlett, D. H. (2008). Prokaryotic lifestyles in deep sea habitats. Extremophiles 12, 15–25. doi: 10.1007/s00792-006-0059-5
Li, R., Tun, H. M., Jahan, M., Zhang, Z., Kumar, A., Fernando, D., et al. (2017). Comparison of DNA-, PMA-, and RNA-based 16S rRNA Illumina sequencing for detection of live bacteria in water. Sci. Rep . 7:5752. doi: 10.1038/s41598-017-02516-3
McMurdie, P. J., and Holmes, S. (2013). Phyloseq: an R package for reproducible interactive analysis and graphics of microbiome census data. PLoS ONE 8:e61217. doi: 10.1371/journal.pone.0061217
McNeil, C. L., and D'Asaro, E. A. (2014). A calibration equation for oxygen optodes based on physical properties of the sensing foil. Limnol. Oceanogr. Methods 12, 139–154. doi: 10.4319/lom.2014.12.139
McNichol, J., Sylva, S. P., Thomas, F., Taylor, C. D., Sievert, S. M., and Seewald, J. S. (2016). Assessing microbial processes in deep-sea hydrothermal systems by incubation at in situ temperature and pressure. Deep. Res. Part I 115, 221–232. doi: 10.1016/j.dsr.2016.06.011
Moran, M. A., González, J. M., and Kiene, R. P. (2003). Linking a bacterial taxon to sulfur cycling in the sea: studies of the marine Roseobacter group. Geomicrobiol. J . 20, 375–388. doi: 10.1080/01490450303901
Nogi, Y., Hosoya, S., Kato, C., and Horikoshi, K. (2004). Colwellia piezophila sp. nov. a novel piezophilic species from deep-sea sediments of the Japan Trench. Int. J. Syst. Evol. Microbiol . 54, 1627–1631. doi: 10.1099/ijs.0.03049-0
Orsi, W. D., Smith, J. M., Liu, S., Liu, Z., Sakamoto, C. M., Wilken, S., et al. (2016). Diverse, uncultivated bacteria and archaea underlying the cycling of dissolved protein in the ocean. ISME J . 10, 2158–2173. doi: 10.1038/ismej.2016.20
Parada, A. E., Needham, D. M., and Fuhrman, J. A. (2016). Every base matters: assessing small subunit rRNA primers for marine microbiomes with mock communities, time series and global field samples. Environ. Microbiol . 18, 1403–1414. doi: 10.1111/1462-2920.13023
Parkes, R. J., Sellek, G., Webster, G., Martin, D., Anders, E., Weightman, A. J., et al. (2009). Culturable prokaryotic diversity of deep, gas hydrate sediments: first use of a continuous high-pressure, anaerobic, enrichment and isolation system for subseafloor sediments (DeepIsoBUG). Environ. Microbiol . 11, 3140–3153. doi: 10.1111/j.1462-2920.2009.02018.x
Peoples, L., Norenberg, M., Price, D., McGoldrick, M., Novotny, M., Bochdansky, A., et al. (2019). A full-ocean-depth rated modular lander and pressure-retaining sampler capable of collecting hadal-endemic microbes under in situ conditions. Deep Sea Res. Part I 143, 50–57. doi: 10.1016/j.dsr.2018.11.010
Quast, C., Pruesse, E., Yilmaz, P., Gerken, J., Schweer, T., Yarza, P., et al. (2013). The SILVA ribosomal RNA gene database project: Improved data processing and web-based tools. Nucleic Acids Res . 41, D590–D596. doi: 10.1093/nar/gks1219
R Core Team (2008). R Software . R Found. Stat. Comput.
Ramirez-Llodra, E., Brandt, A., Danovaro, R., De Mol, B., Escobar, E., German, C. R., et al. (2010). Deep, diverse and definitely different: unique attributes of the world's largest ecosystem. Biogeosciences 7, 2851–2899. doi: 10.5194/bg-7-2851-2010
Ravaux, J., Hamel, G., Zbinden, M., Tasiemski, A. A., Boutet, I., Léger, N., et al. (2013). Thermal limit for metazoan life in question: in vivo heat tolerance of the pompeii worm. PLoS ONE 8:e64074. doi: 10.1371/journal.pone.0064074
Riou, V., Para, J., Garel, M., Guigue, C., Al Ali, B., Santinelli, C., et al. (2017). Biodegradation of Emiliania huxleyi aggregates by a natural Mediterranean prokaryotic community under increasing hydrostatic pressure. Prog. Oceanogr . 163, 271–281. doi: 10.1016/j.pocean.2017.01.005
Shillito, B., Gaill, F., and Ravaux, J. (2014). The ipocamp pressure incubator for deep-sea fauna. J. Mar. Sci. Technol . 22, 97–102. doi: 10.6119/JMST-013-0718-3
Shillito, B., Hamel, G., Duchi, C., Cottin, D., Sarrazin, J., Sarradin, P. M., et al. (2008). Live capture of megafauna from 2300m depth, using a newly designed pressurized recovery device. Deep Sea Res. Part I 55, 881–889. doi: 10.1016/j.dsr.2008.03.010
Simon, M., and Azam, F. (1989). Protein content and protein synthesis rates of planktonic marine bacteria. Mar. Ecol. Prog. Ser . 51, 201–213. doi: 10.3354/meps051201
Tabor, P., and Colwell, R. R. (1976). “Initial investigation with a deep ocean in situ sampler,” in In Proceedings of the MTS/ IEEE OCEANS'76. IEEE , (Washington, DC), 13D−1–13D−4.
Tamburini, C. (2006). “Life under pressure. Deep-sea microbial ecology,” in Life as we know it. Series: Cellular Origin and Life in Extreme Habitats and Astrobiology , vol. 10. ed J. Seckbach (Dordrecht: Springer), 650.
Tamburini, C., Boutrif, M., Garel, M., Colwell, R. R., and Deming, J. W. (2013). Prokaryotic responses to hydrostatic pressure in the ocean - a review. Environ. Microbiol . 15, 1262–1274. doi: 10.1111/1462-2920.12084
Tamburini, C., Garcin, J., and Bianchi, A. (2003). Role of deep-sea bacteria in organic matter mineralization and adaptation to hydrostatic pressure conditions in the NW Mediterranean Sea. Aquat. Microb. Ecol . 32, 209–218. doi: 10.3354/ame032209
Tamburini, C., Garcin, J., Grégori, G., Leblanc, K., Rimmelin, P., and Kirchman, D. L. (2006). Pressure effects on surface Mediterranean prokaryotes and biogenic silica dissolution during a diatom sinking experiment. Aquat. Microb . 43, 267–276. doi: 10.3354/ame043267
Tamburini, C., Garcin, J., Ragot, M., and Bianchi, A. (2002). Biopolymer hydrolysis and bacterial production under ambient hydrostatic pressure through a 2000 m water column in the NW Mediterranean. Deep Sea Res. Part II 49, 2109–2123. doi: 10.1016/S0967-0645(02)00030-9
Tamburini, C., Garel, M., Al Ali, B., Mérigot, B., Kriwy, P., Charrière, B., et al. (2009a). Distribution and activity of bacteria and archaea in the different water masses of the Tyrrhenian Sea. Deep Sea Res. Part II 56, 700–712. doi: 10.1016/j.dsr2.2008.07.021
Tamburini, C., Goutx, M., Guigue, C., Garel, M., Lefèvre, D., Charrière, B., et al. (2009b). Effects of hydrostatic pressure on microbial alteration of sinking fecal pellets. Deep Sea Res. Part II Top. Stud. Oceanogr. 56, 1533–1546. doi: 10.1016/j.dsr2.2008.12.035
Teira, E., Lebaron, P., van Aken, H., and Herndl, G. J. (2006). Distribution and activity of bacteria and Archaea in the deep water masses of the North Atlantic. Limnol. Oceanogr . 51, 2131–2144. doi: 10.4319/lo.2006.51.5.2131
Vezzi, A., Campanaro, S., D'Angelo, M., Simonato, F., Vitulo, N., Lauro, F. M., et al. (2005). Life at depth: photobacterium profundum genome sequence and expression analysis. Science 307, 1459–1461. doi: 10.1126/science.1103341
Wannicke, N., Frindte, K., Gust, G., Liskow, I., Wacker, A., Meyer, A., et al. (2015). Measuring bacterial activity and community composition at high hydrostatic pressure using a novel experimental approach: a pilot study. FEMS Microbiol. Ecol . 91, 1–15. doi: 10.1093/femsec/fiv036
Whitman, W. B., Coleman, D. C., and Wiebe, W. J. (1998). Perspective prokaryotes: the unseen majority. Proc. Natl. Acad. Sci. U.S.A. 95, 6578–6583.
Keywords: pressure-retaining sampler, deep sea, in situ sampling, prokaryotic activities, prokaryotic diversity
Citation: Garel M, Bonin P, Martini S, Guasco S, Roumagnac M, Bhairy N, Armougom F and Tamburini C (2019) Pressure-Retaining Sampler and High-Pressure Systems to Study Deep-Sea Microbes Under in situ Conditions. Front. Microbiol . 10:453. doi: 10.3389/fmicb.2019.00453
Received: 07 December 2018; Accepted: 20 February 2019; Published: 09 April 2019.
Reviewed by:
Copyright © 2019 Garel, Bonin, Martini, Guasco, Roumagnac, Bhairy, Armougom and Tamburini. This is an open-access article distributed under the terms of the Creative Commons Attribution License (CC BY) . The use, distribution or reproduction in other forums is permitted, provided the original author(s) and the copyright owner(s) are credited and that the original publication in this journal is cited, in accordance with accepted academic practice. No use, distribution or reproduction is permitted which does not comply with these terms.
*Correspondence: Christian Tamburini, [email protected]
Disclaimer: All claims expressed in this article are solely those of the authors and do not necessarily represent those of their affiliated organizations, or those of the publisher, the editors and the reviewers. Any product that may be evaluated in this article or claim that may be made by its manufacturer is not guaranteed or endorsed by the publisher.
- DOI: 10.1109/OCEANS.2014.7002998
- Corpus ID: 22007129
7000M pressure experiment of a deep-sea hydraulic manipulator system
- Qifeng Zhang , Jun Chen , +5 authors Yuangui Tang
- Published in Oceans 1 September 2014
- Engineering, Environmental Science
Figures and Tables from this paper
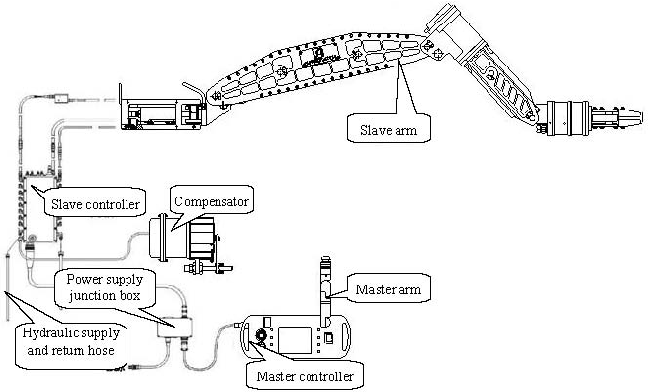
9 Citations
Experiment research on control performance for the actuators of a deep-sea hydraulic manipulator, influence of ambient pressure on performance of a deep-sea hydraulic manipulator, development of a full ocean depth hydraulic manipulator system, autonomous underwater vehicle (auv) based on oil-filled modular controller, preliminary experimental research on the influence of counterbalance valves on the operation of a heavy hydraulic manipulator during long-range straight-line movement, a computation effective singularity avoidance method for the underwater manipulator with a non-spherical wrist, underwater manipulators: a review, a compliant-rigid underwater manipulator for enhanced safety and precision performance, underwater robot manipulation: advances, challenges and prospective ventures, 13 references, design and experiments of a deep-sea hydraulic manipulator system, development of a 7-function hydraulic underwater manipulator system, development of a deep ocean master-slave electric manipulator control system, hybrid position/force control of a hydraulic underwater manipulator, workspace control system of underwater tele-operated manipulators on rovs, control of underwater manipulators mounted on an rov using base force information, dynamic workspace control method for underwater manipulator of floating rov, a method of inverse kinematics of a 7-function underwater hydraulic manipulator, model reference adaptive control for a hydraulic underwater manipulator, analysis on dynamics of underwater robot manipulators based on iterative learning control and time-scale transformation, related papers.
Showing 1 through 3 of 0 Related Papers
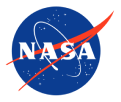
Suggested Searches
- Climate Change
- Expedition 64
- Mars perseverance
- SpaceX Crew-2
- International Space Station
- View All Topics A-Z
Humans in Space
Earth & climate, the solar system, the universe, aeronautics, learning resources, news & events.
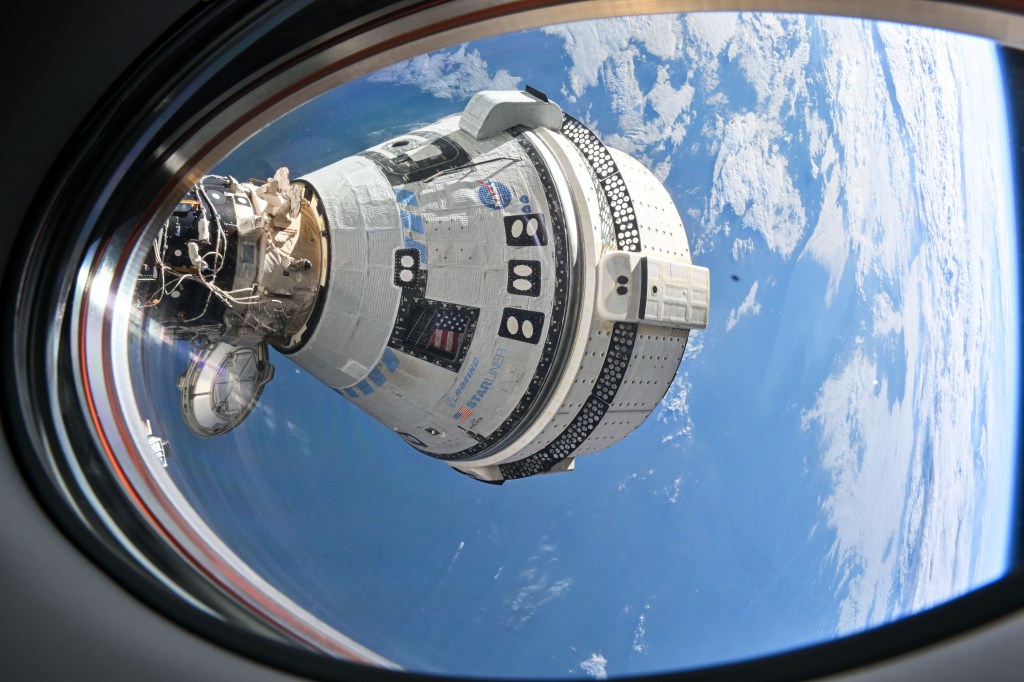

FAQ: NASA’s Boeing Crew Flight Test Return Status

Danish Instrument Helps NASA’s Juno Spacecraft See Radiation
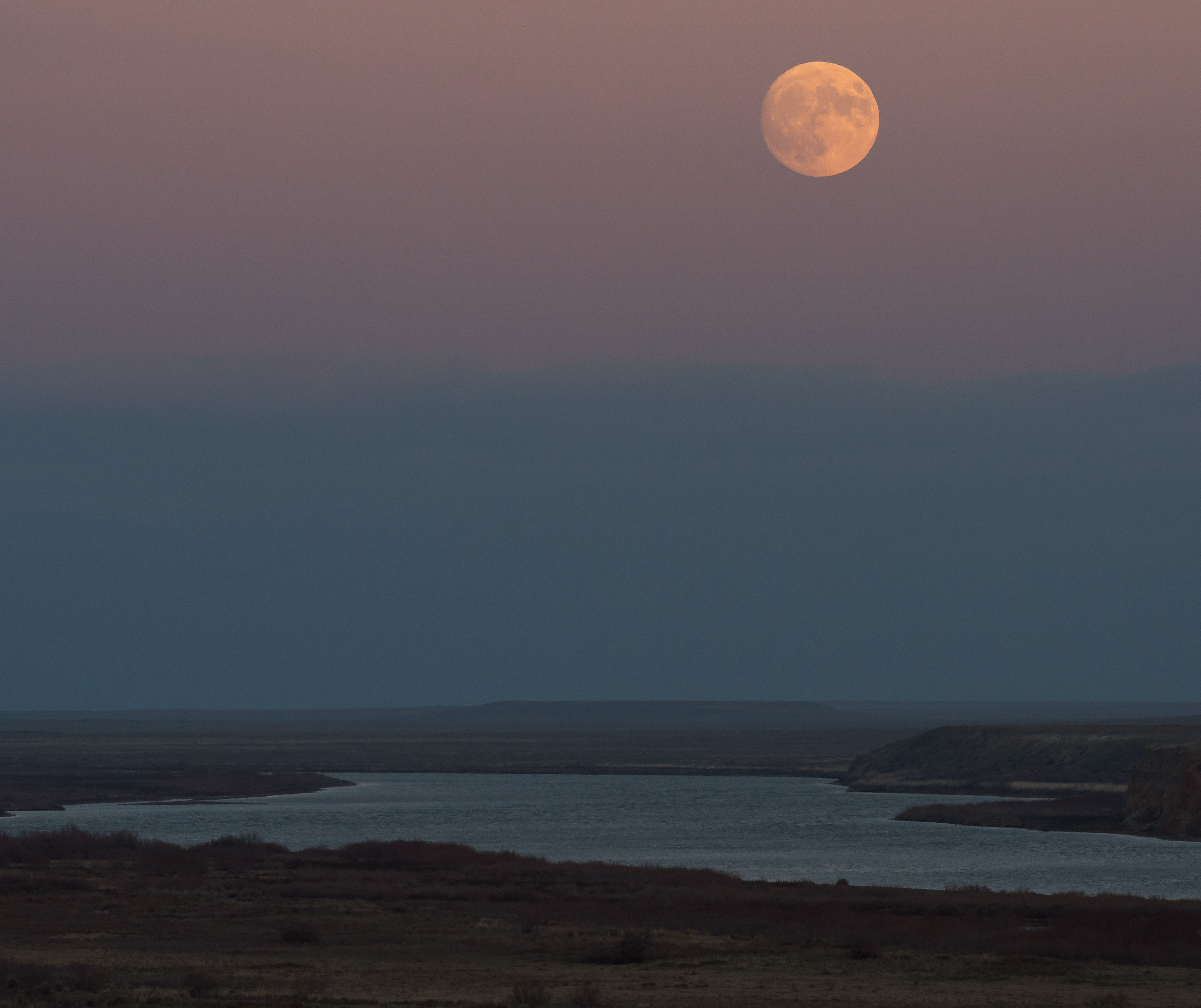
Super Blue Moons: Your Questions Answered
- Search All NASA Missions
- A to Z List of Missions
- Upcoming Launches and Landings
- Spaceships and Rockets
- Communicating with Missions
- James Webb Space Telescope
- Hubble Space Telescope
- Why Go to Space
- Commercial Space
- Destinations
- Living in Space
- Explore Earth Science
- Earth, Our Planet
- Earth Science in Action
- Earth Multimedia
- Earth Science Researchers
- Pluto & Dwarf Planets
- Asteroids, Comets & Meteors
- The Kuiper Belt
- The Oort Cloud
- Skywatching
- The Search for Life in the Universe
- Black Holes
- The Big Bang
- Dark Energy & Dark Matter
- Earth Science
- Planetary Science
- Astrophysics & Space Science
- The Sun & Heliophysics
- Biological & Physical Sciences
- Lunar Science
- Citizen Science
- Astromaterials
- Aeronautics Research
- Human Space Travel Research
- Science in the Air
- NASA Aircraft
- Flight Innovation
- Supersonic Flight
- Air Traffic Solutions
- Green Aviation Tech
- Drones & You
- Technology Transfer & Spinoffs
- Space Travel Technology
- Technology Living in Space
- Manufacturing and Materials
- Science Instruments
- For Kids and Students
- For Educators
- For Colleges and Universities
- For Professionals
- Science for Everyone
- Requests for Exhibits, Artifacts, or Speakers
- STEM Engagement at NASA
- NASA's Impacts
- Centers and Facilities
- Directorates
- Organizations
- People of NASA
- Internships
- Our History
- Doing Business with NASA
- Get Involved
NASA en Español
- Aeronáutica
- Ciencias Terrestres
- Sistema Solar
- All NASA News
- Video Series on NASA+
- Newsletters
- Social Media
- Media Resources
- Upcoming Launches & Landings
- Virtual Events
- Sounds and Ringtones
- Interactives
- STEM Multimedia
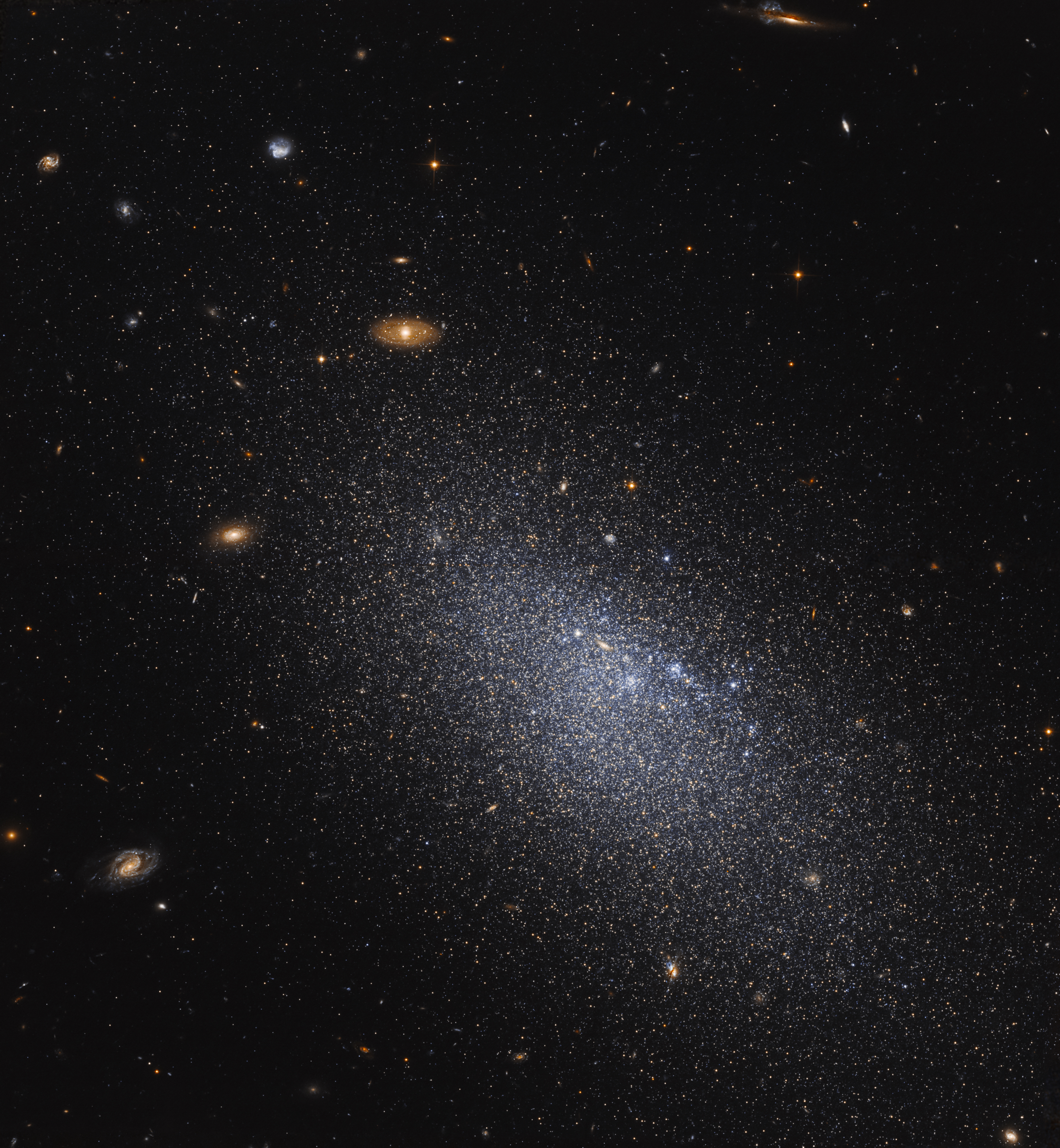
Hubble Examines a Possible Relic

NextSTEP R: Lunar Logistics and Mobility Studies
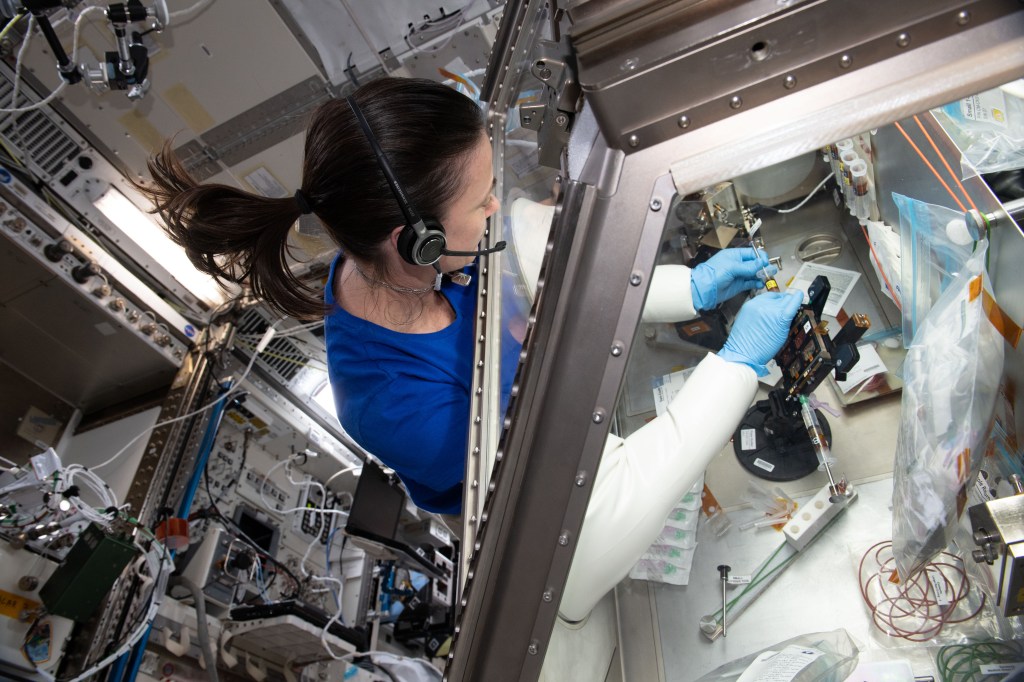
Station Science Top News: August 16, 2024
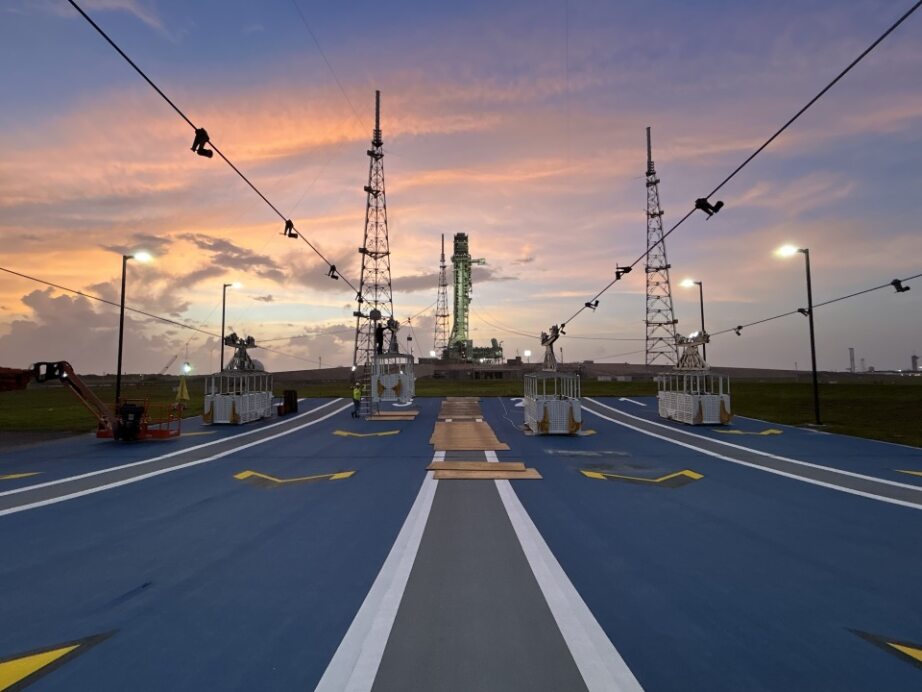
Artemis Emergency Egress System Emphasizes Crew Safety
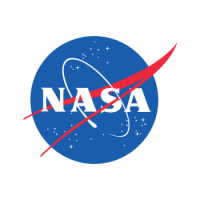
STV Precursor Coincident Datasets
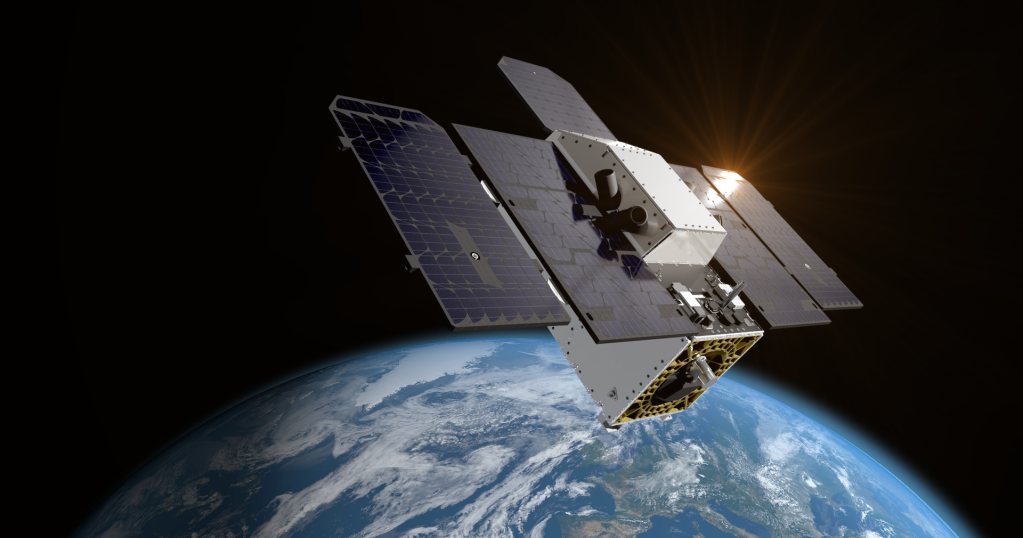
NASA-Designed Greenhouse Gas-Detection Instrument Launches
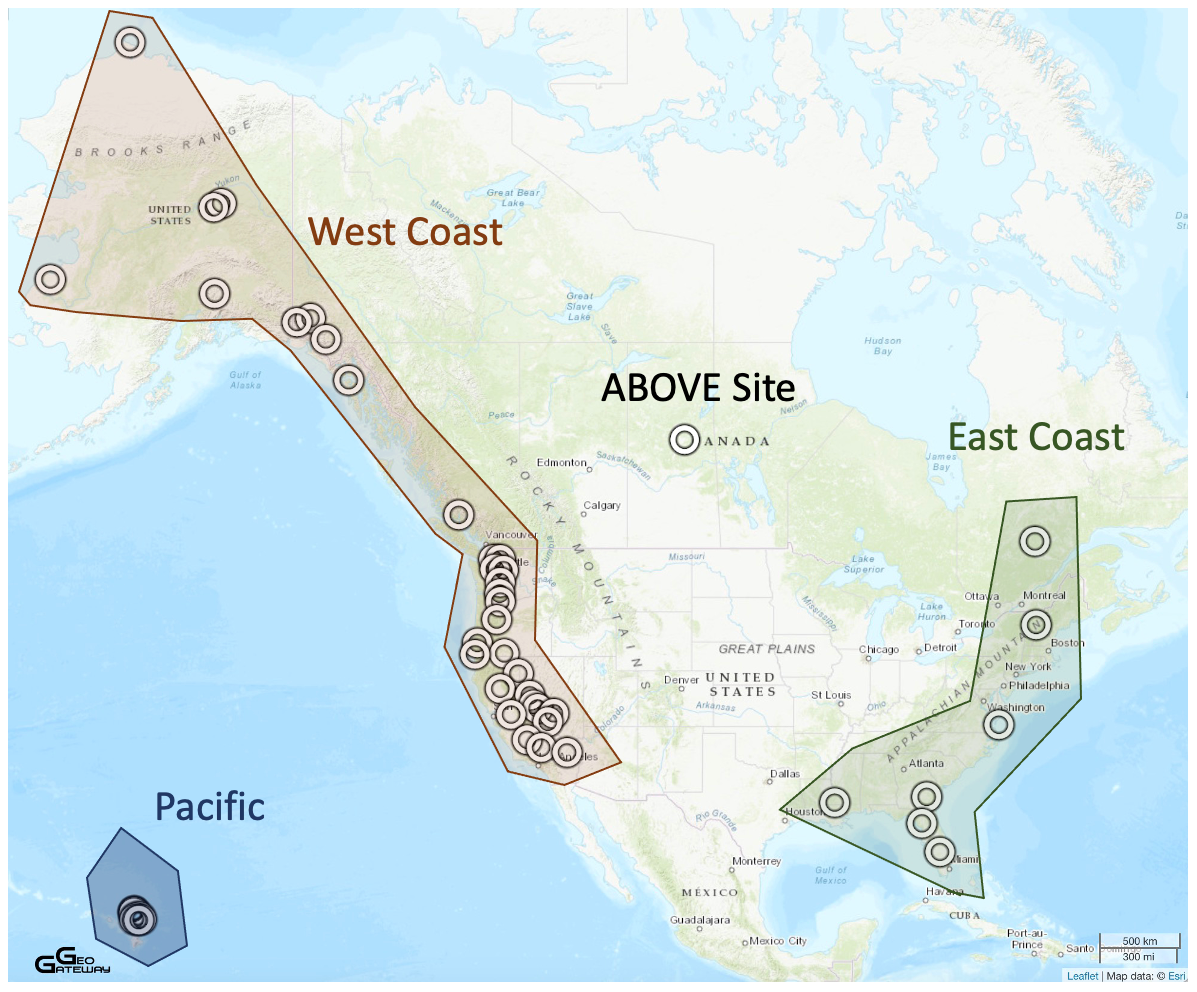
Airborne Surface, Cryosphere, Ecosystem, and Nearshore Topography
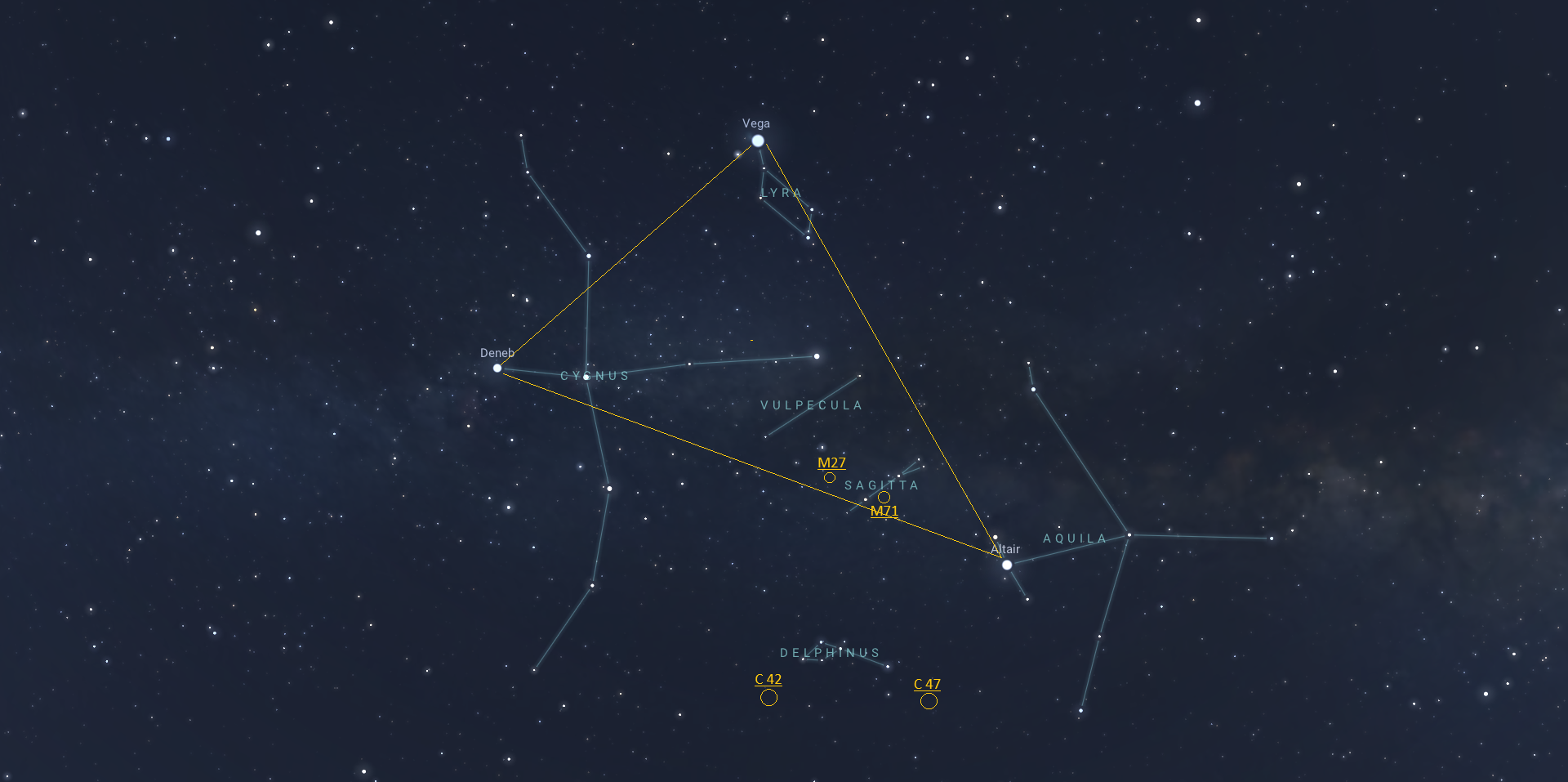
The Summer Triangle’s Hidden Treasures
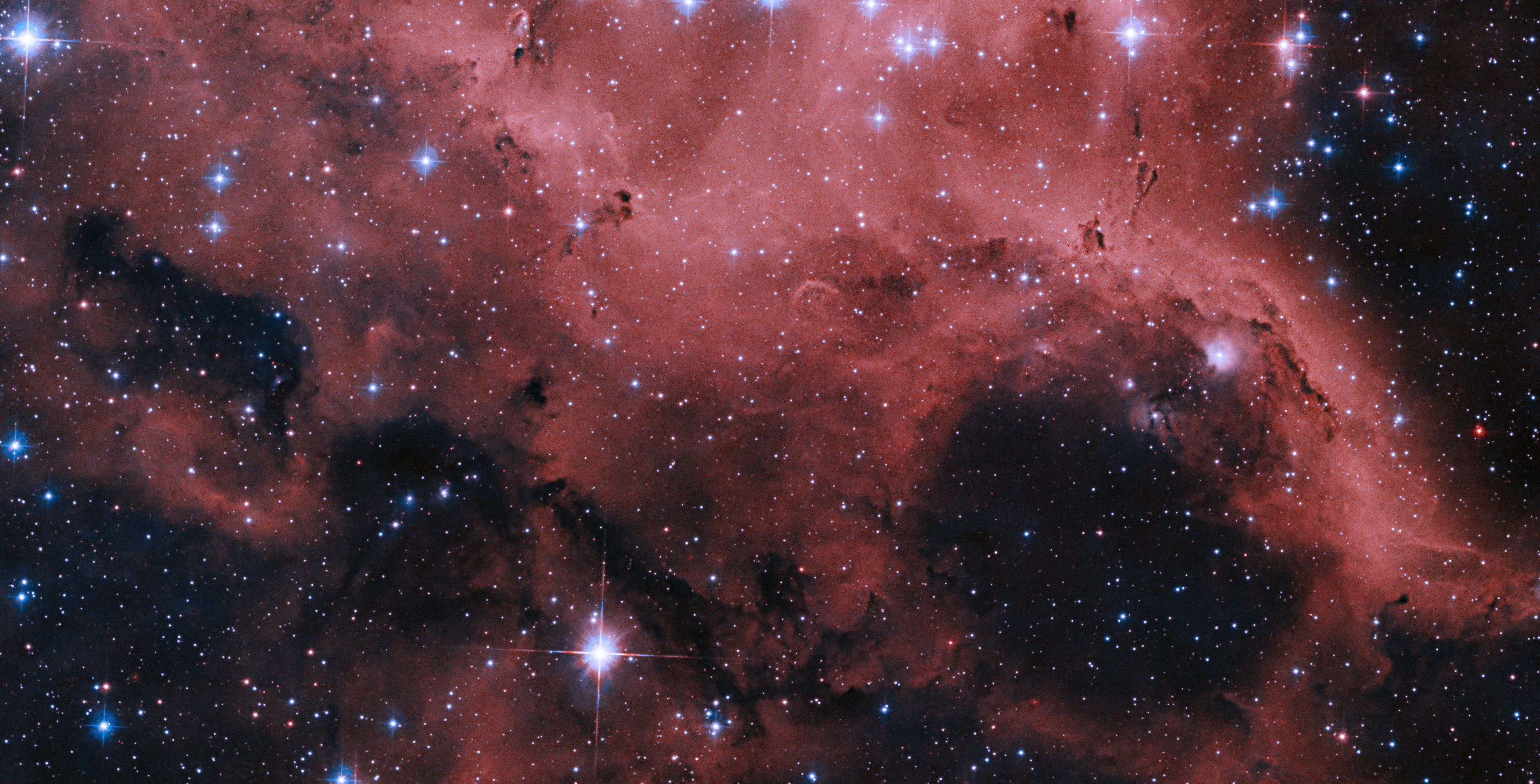
Hubble Spots Billowing Bubbles of Stellar Floss
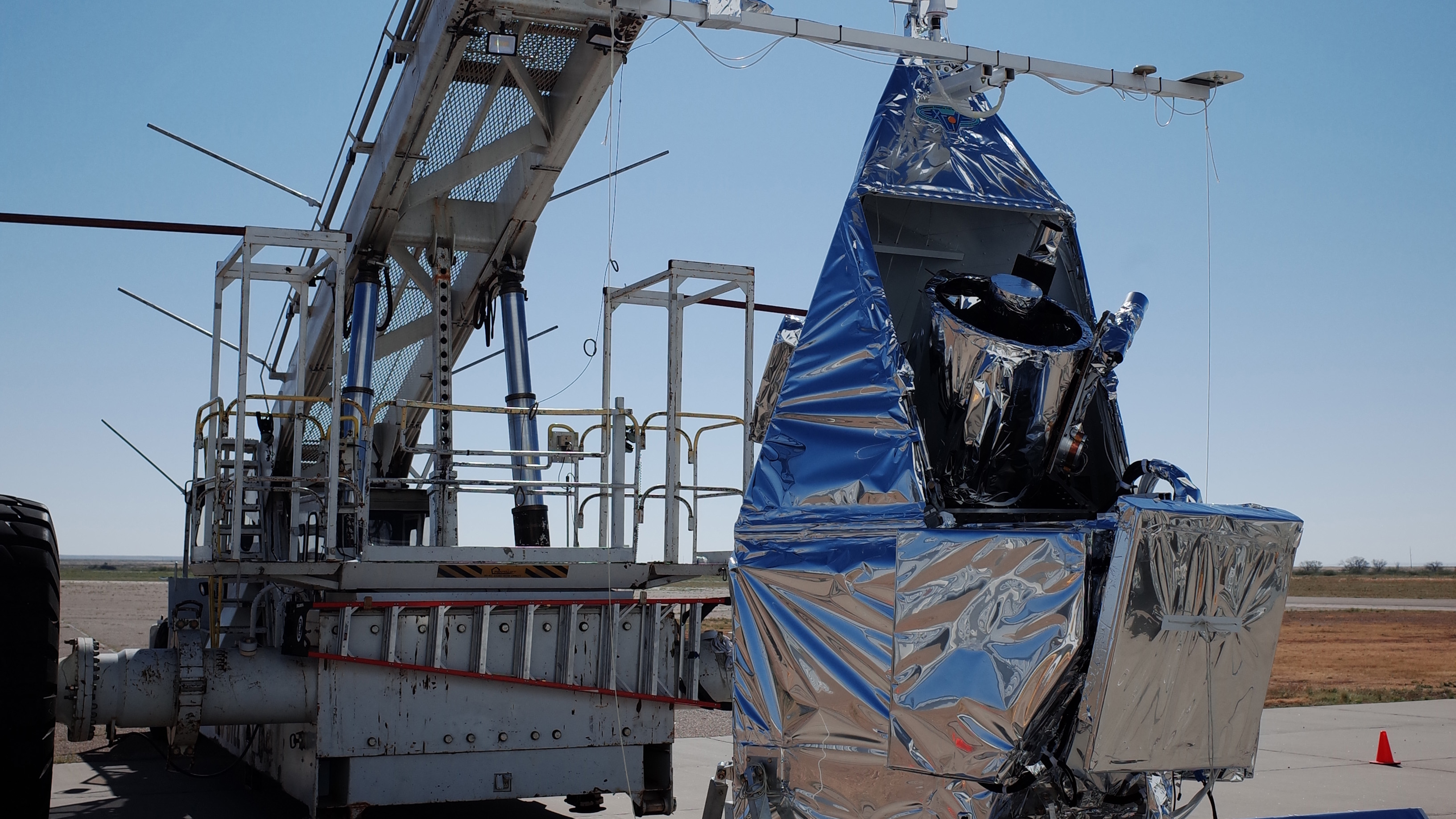
EXCITE (EXoplanet Climate Infrared TElescope)

Entrepreneurs Challenge Prize Winner Uses Artificial Intelligence to Identify Methane Emissions

NASA Celebrates Ames’s Legacy of Research on National Aviation Day

At Work and Beyond, NASA Employees Find Joy in Aviation
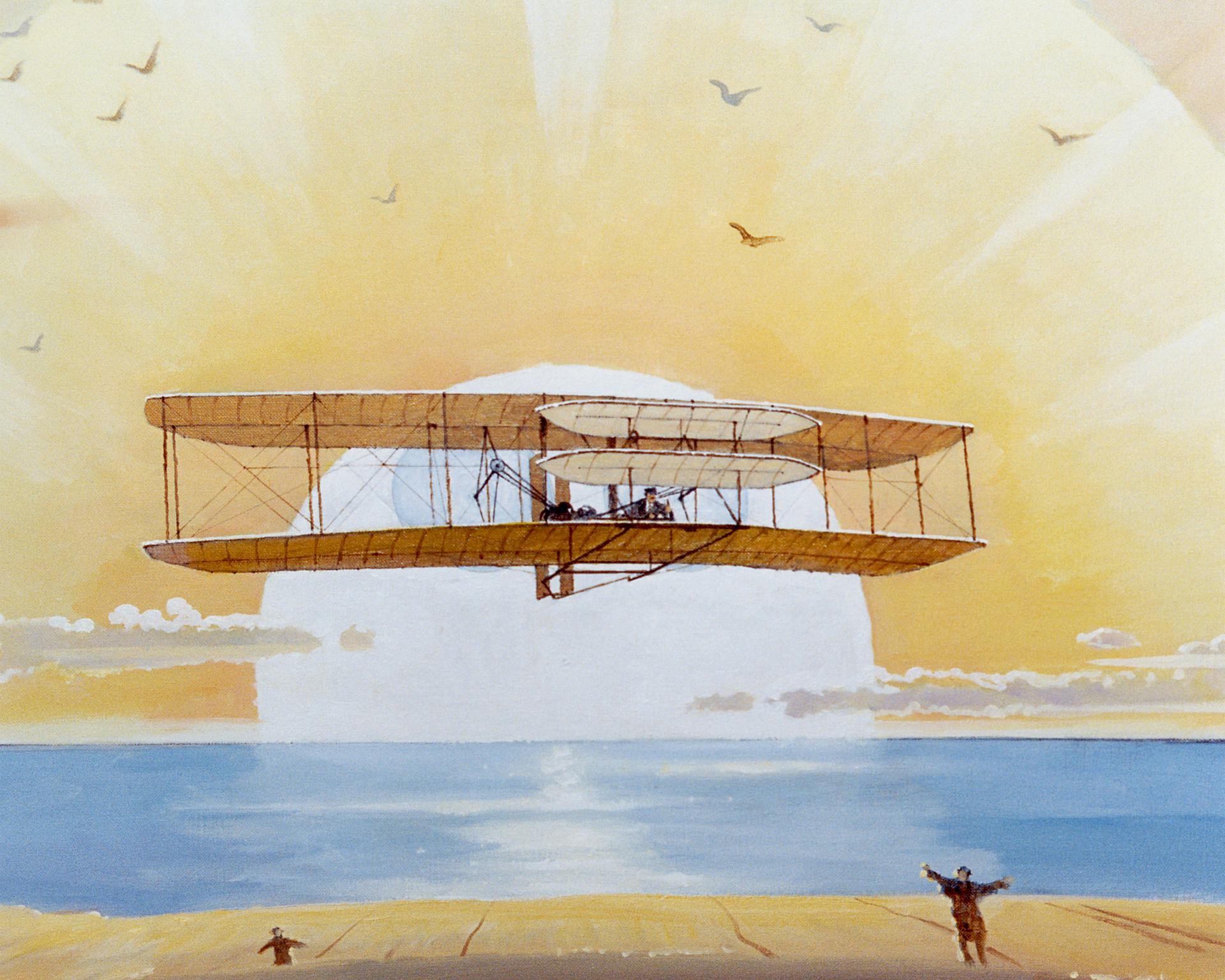
Orville Wright and National Aviation Day
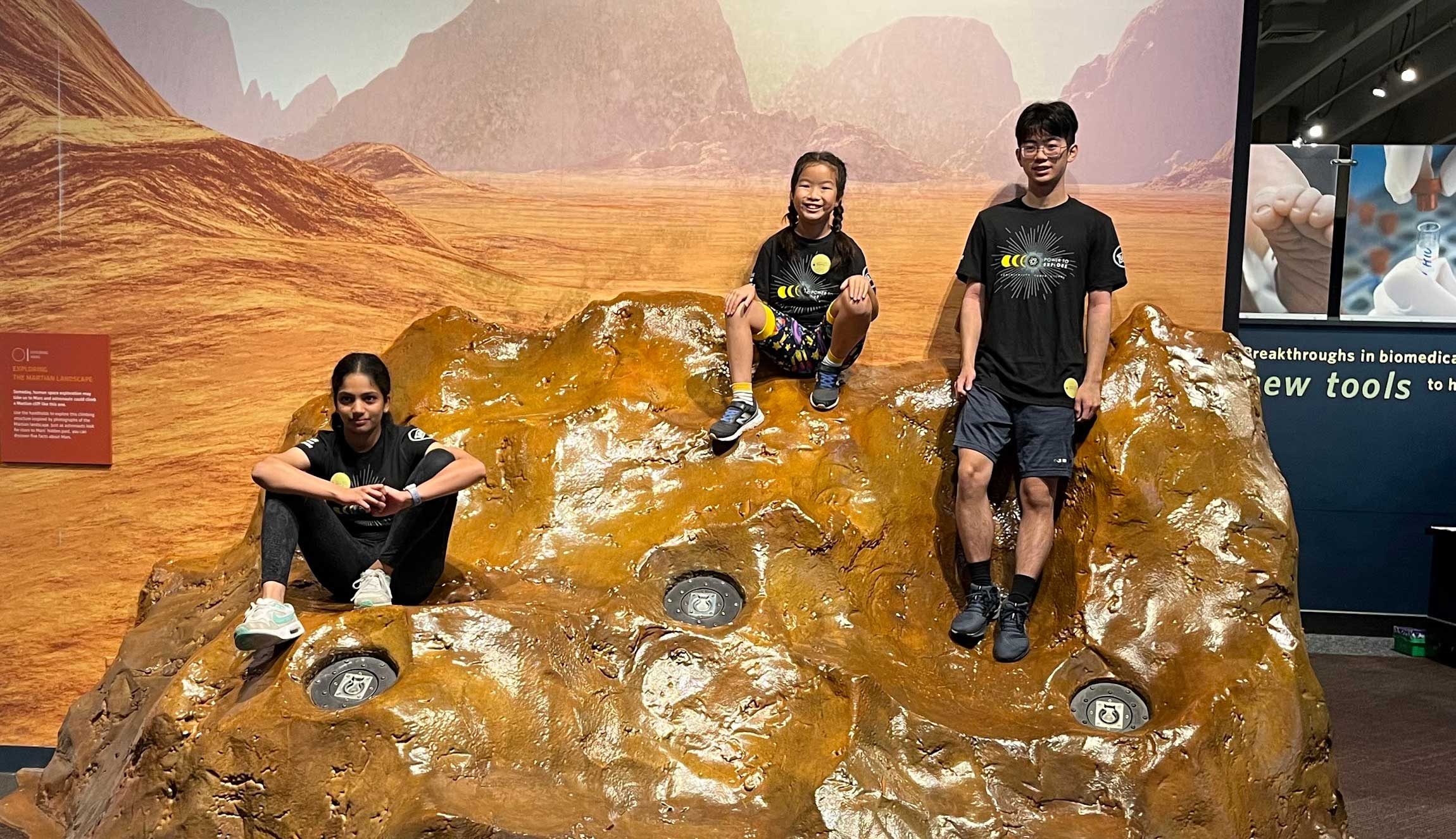
Perseverance Pays Off for Student Challenge Winners
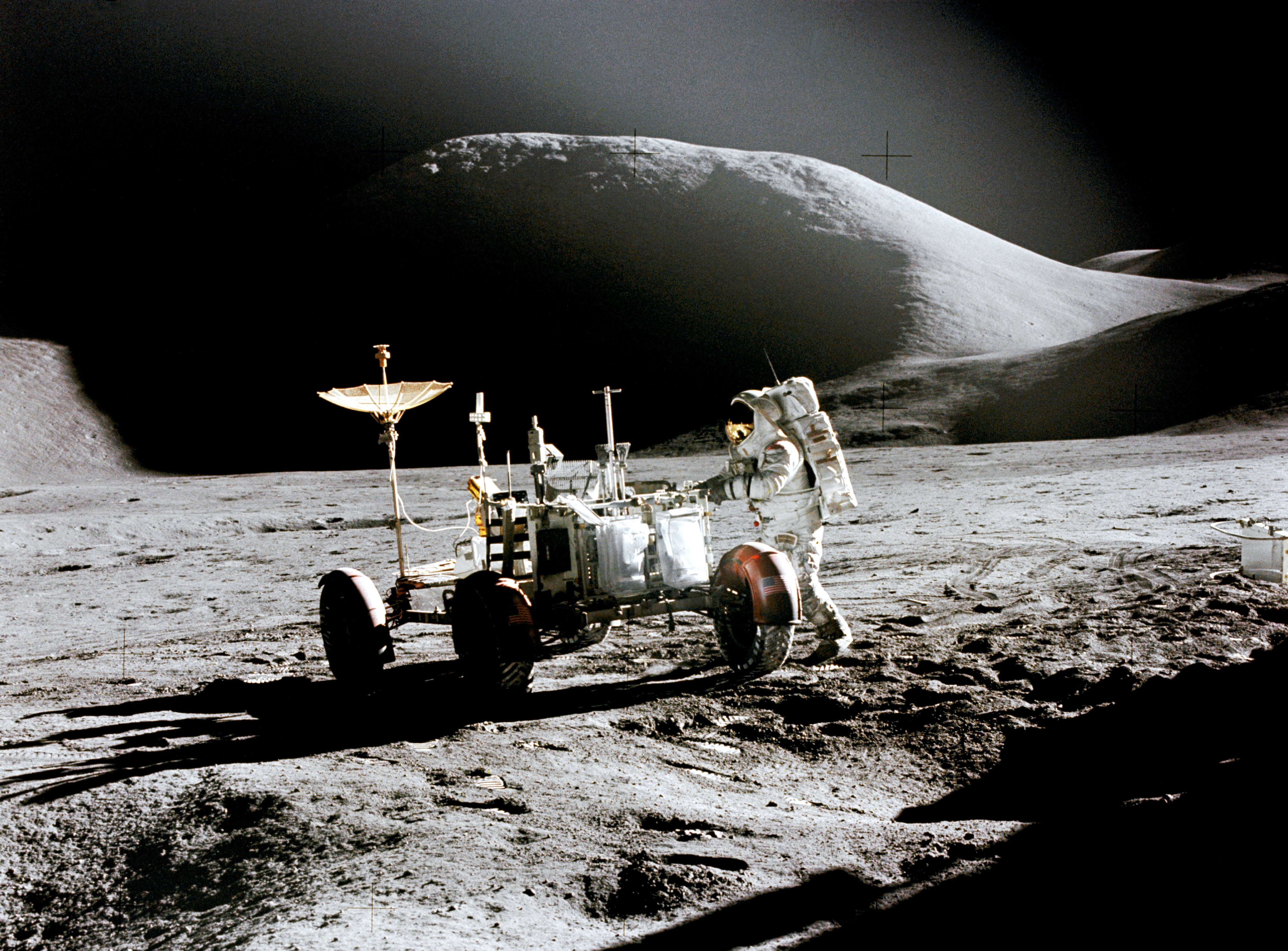
Amendment 41: DRAFT F.13 Lunar Terrain Vehicle Instruments Program Released for Community Comment.
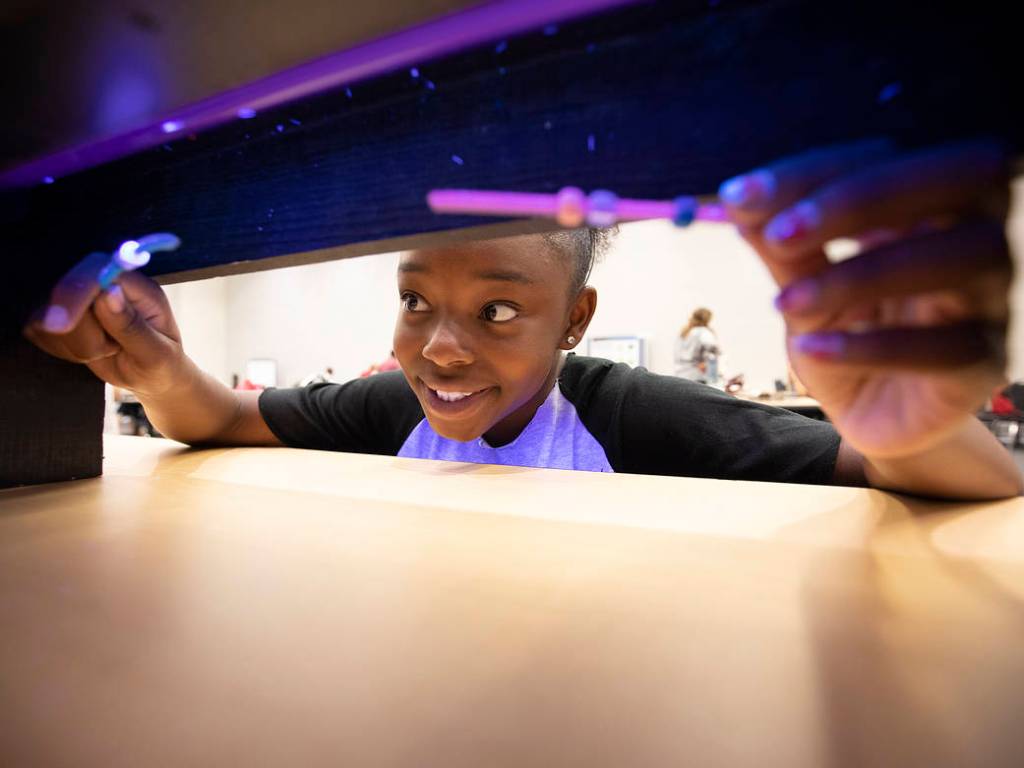
How Do I Navigate NASA Learning Resources and Opportunities?

55 Years Ago: Apollo 11 Astronauts End Quarantine, Feted from Coast to Coast
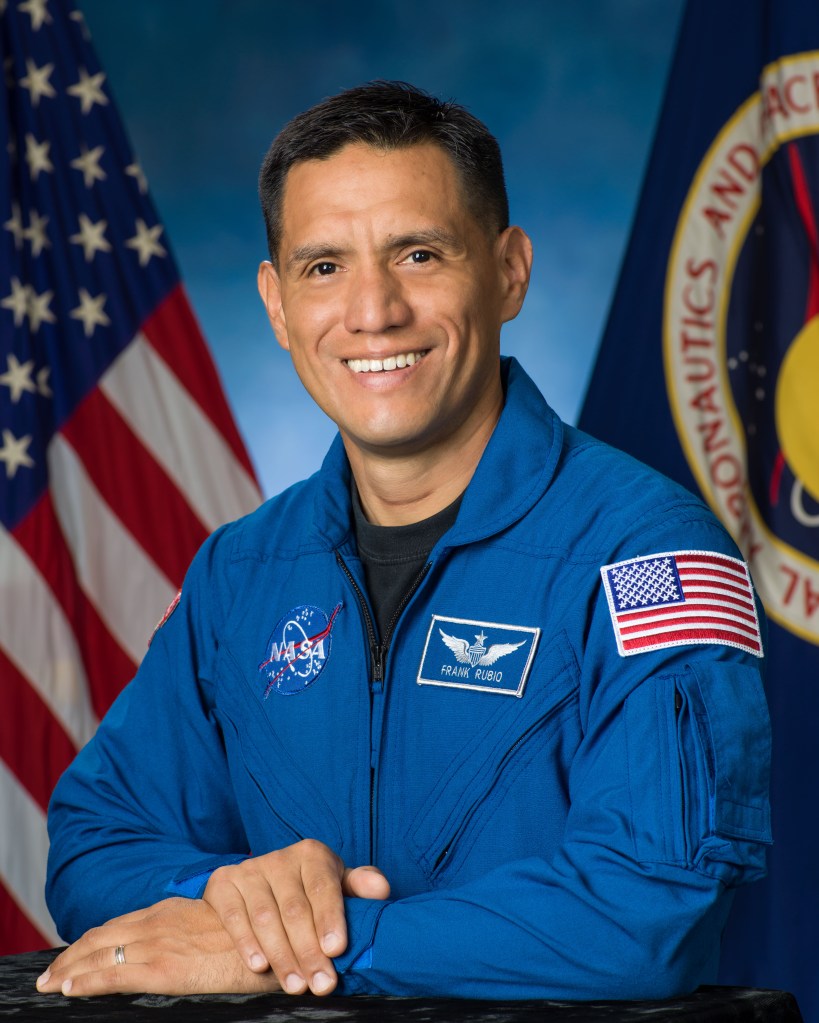
Astronauta de la NASA Frank Rubio
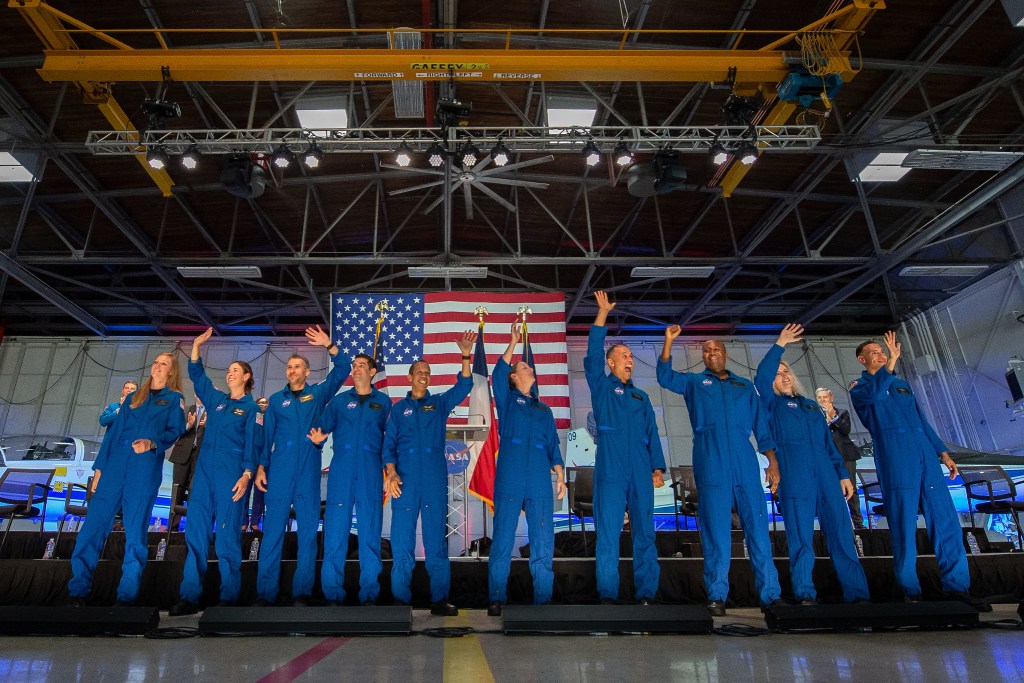
Diez maneras en que los estudiantes pueden prepararse para ser astronautas
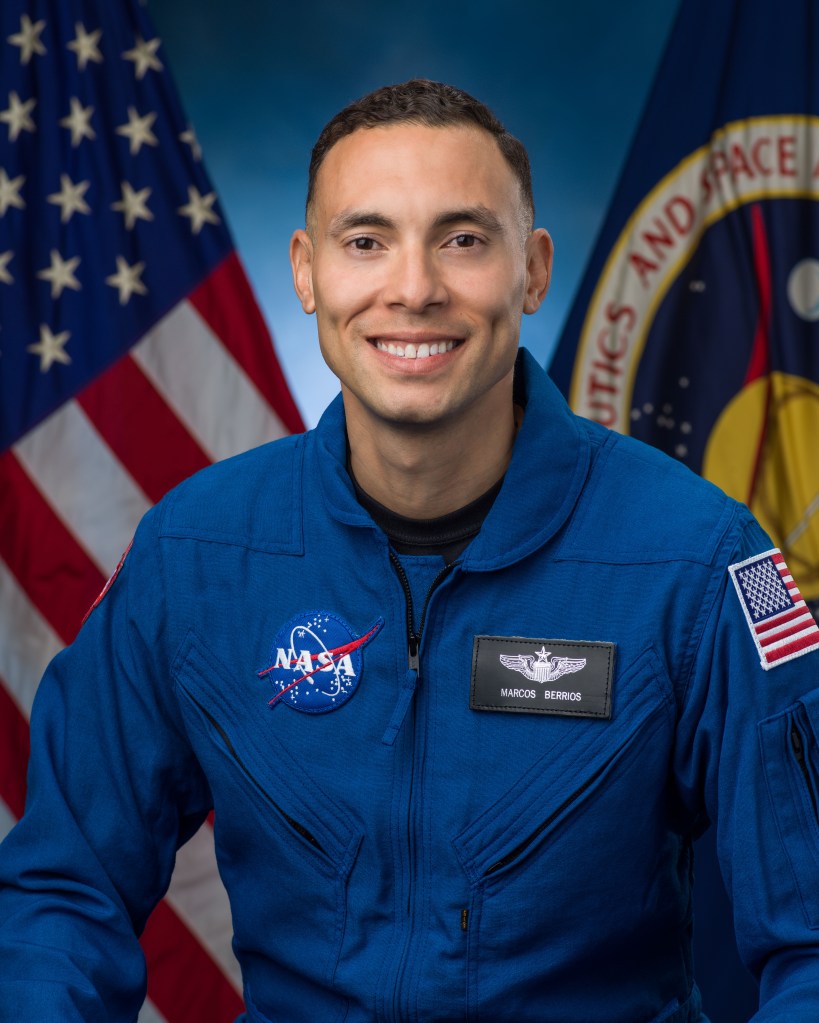
Astronauta de la NASA Marcos Berríos
Simulating early ocean vents shows life’s building blocks form under pressure, jet propulsion laboratory, under the ancient sea, signs of life.
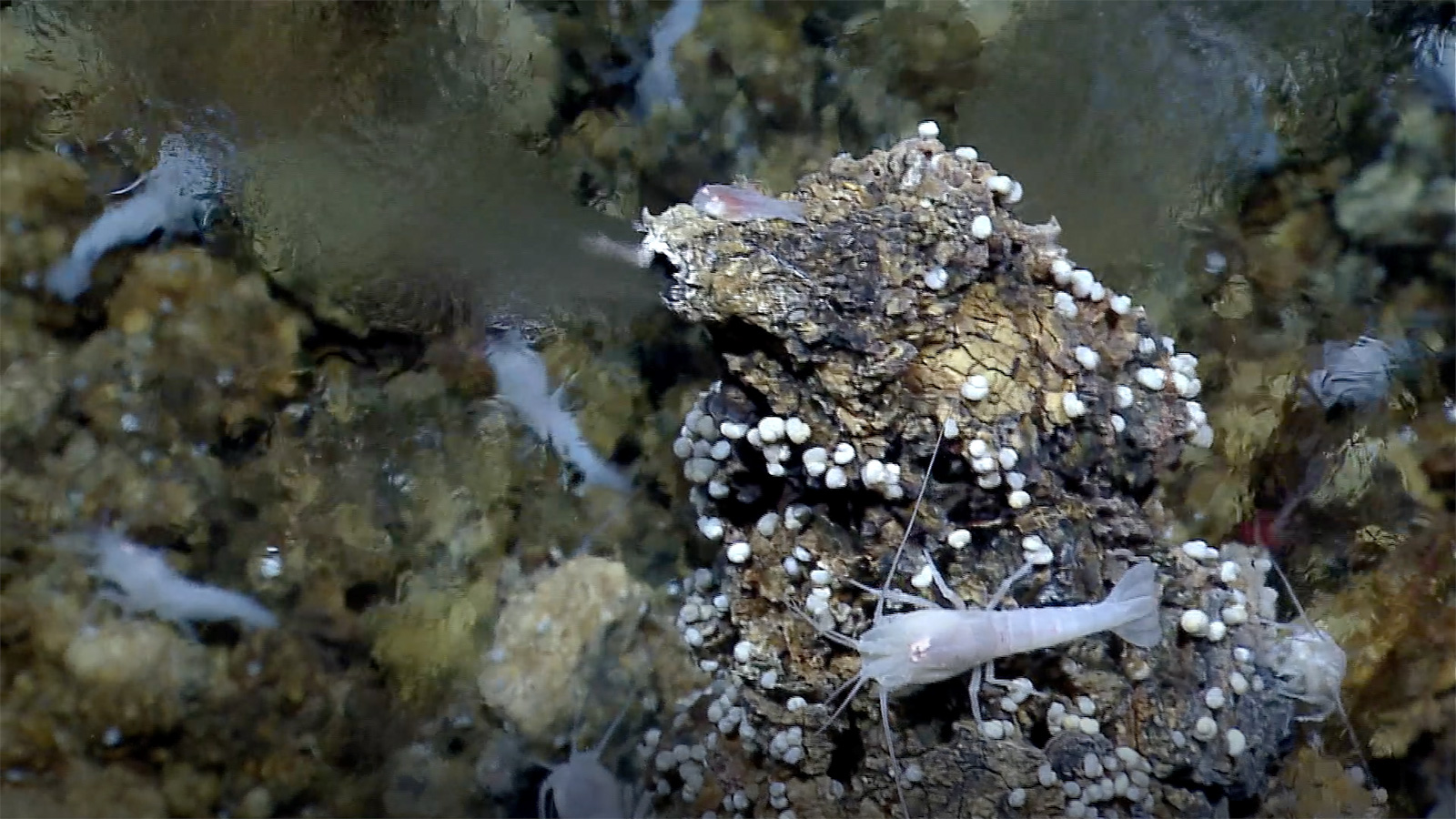
Where did life first form on Earth? Some scientists think it could have been around hydrothermal vents that may have existed at the bottom of the ocean 4.5 billion years ago. In a new paper in the journal Astrobiology, scientists at NASA’s Jet Propulsion Laboratory describe how they mimicked possible ancient undersea environments with a complex experimental setup. They showed that under extreme pressure, fluid from these ancient seafloor cracks mixed with ocean water could have reacted with minerals from the hydrothermal vents to produce organic molecules — the building blocks that compose nearly all life on Earth.
In particular, the research lays important groundwork for in-depth studies of such ocean worlds as Saturn’s moon Enceladus and Jupiter’s moon Europa, which are both thought to have liquid-water oceans buried beneath thick icy crusts and may host hydrothermal activity similar to what’s being simulated at JPL. This area of research belongs to a field of study known as astrobiology, and the work was done by the JPL Icy Worlds team as part of the former NASA Astrobiology Institute.
To simulate conditions that might have existed on the ocean floor of a newly formed Earth, before the sea teemed with life, then-graduate student Lauren White and colleagues conducted an experiment that brought together three key ingredients: hydrogen-rich water, like the kind that could have flowed out from beneath the seafloor through vents; seawater enriched with carbon dioxide, as it would have been from the ancient atmosphere; and a few minerals that might have formed in that environment.
White and colleagues — including her graduate advisor, retired JPL scientist Michael Russell — simulated vents that didn’t spew particularly hot water (it was only about 212 Fahrenheit, or 100 degrees Celsius). One major challenge with creating the experimental setup was maintaining the same pressure found 0.6 miles (1 kilometers) below the ocean surface — about 100 times the air pressure at sea level. Previous experiments have tested similar chemical reactions in individual high-pressure chambers, but White and her colleagues wanted to more fully replicate the physical properties of these environments, including the way the fluids flow and mix together. This would require maintaining the high pressure in multiple chambers, which added to the complexity of the project. (Because a crack or leak in even a single high-pressure chamber poses the threat of an explosion, it’s standard operating procedure in such cases to install a blast shield between the apparatus and the scientists.)
The scientists wanted to determine whether such ancient conditions could have produced organic molecules — those containing carbon atoms in loops or chains, as well as with other atoms, most commonly hydrogen. Examples of complex organic molecules include amino acids, which can eventually form DNA and RNA.
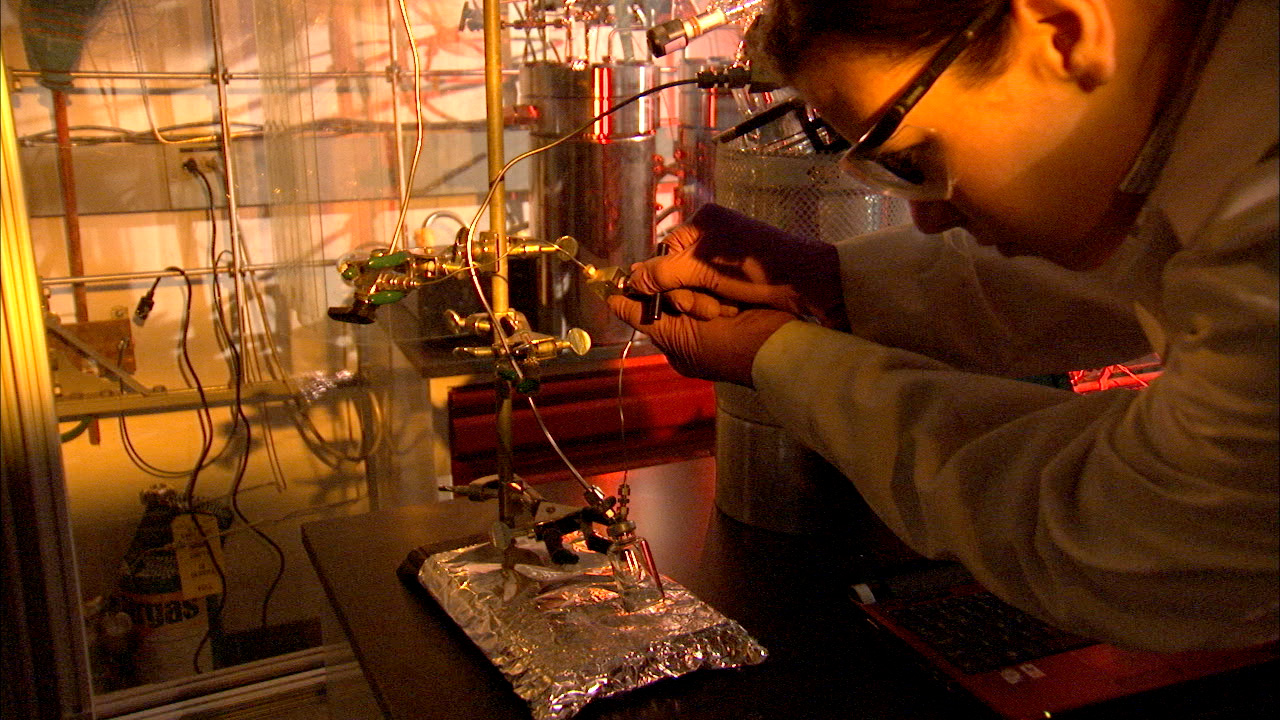
But just as eggs, flour, butter and sugar aren’t the same thing as a cake, the presence of both carbon and hydrogen in the early oceans doesn’t guarantee the formation of organic molecules. While a carbon and a hydrogen atom might reasonably bump into each other in this prehistoric ocean, they wouldn’t automatically join to form an organic compound. That process requires energy, and just like a ball won’t roll up a hill by itself, carbon and hydrogen won’t bind together without an energetic push.
A previous study by White and her colleagues showed that water pulsing through hydrothermal vents could have formed iron sulfides. By acting as a catalyst, iron sulfides could provide that energetic push, lowering the amount of energy required for carbon and hydrogen to react together, and increasing the likelihood they would form organics.
The new experiment tested whether this reaction would have been likely to occur under the physical conditions around ancient seafloor vents, if such vents existed at the time. The answer? Yes. The team created formate and trace amounts of methane, both organic molecules.
Naturally occurring methane on Earth is produced largely by living organisms or through the decay of biological material, including plants and animals. Could methane on other planets also be a sign of biological activity? To use methane to search for life on other worlds, scientists need to understand both its biological and non-biological sources, such as the one identified by White and her colleagues.
“I think it’s really significant that we showed that these reactions take place in the presence of those physical factors, like the pressure and the flow,” said White. “We are still a long way from demonstrating that life could have formed in these environments. But if anyone ever wants to make that case, I think we’ll need to have demonstrated the feasibility of every step of the process; we can’t take anything for granted.”
The work builds on Michael Russell’s hypothesis that life on Earth may have formed at the bottom of Earth’s early ocean. The formation of organic molecules would be a major step in this process. Scientists in the same JPL research group have explored other aspects of this work, such as replicating the chemical conditions in the early ocean to demonstrate how amino acids might form there . However, the new study is unique in the way it re-created the physical conditions of those environments.
In the next few years, NASA will launch Europa Clipper, which will orbit Jupiter and perform multiple flybys of the icy moon Europa. Scientists believe plumes there may spew water into space from the moon’s ocean, which lies beneath about 2 to 20 miles (3 to 30 kilometers) of ice. These plumes could provide information about possible hydrothermal processes at the bottom of the ocean, thought to be about 50 miles (80 kilometers) deep. The new paper contributes to a growing understanding of the chemistry that might take place in oceans other than our own, which will help scientists interpret findings of that mission and others to come.
For more information on astrobiology at NASA, visit:
https://astrobiology.nasa.gov/
Calla Cofield Jet Propulsion Laboratory, Pasadena, Calif. 626-808-2469 [email protected]
IEEE Account
- Change Username/Password
- Update Address
Purchase Details
- Payment Options
- Order History
- View Purchased Documents
Profile Information
- Communications Preferences
- Profession and Education
- Technical Interests
- US & Canada: +1 800 678 4333
- Worldwide: +1 732 981 0060
- Contact & Support
- About IEEE Xplore
- Accessibility
- Terms of Use
- Nondiscrimination Policy
- Privacy & Opting Out of Cookies
A not-for-profit organization, IEEE is the world's largest technical professional organization dedicated to advancing technology for the benefit of humanity. © Copyright 2024 IEEE - All rights reserved. Use of this web site signifies your agreement to the terms and conditions.
share this!
November 9, 2023
This article has been reviewed according to Science X's editorial process and policies . Editors have highlighted the following attributes while ensuring the content's credibility:
fact-checked
peer-reviewed publication
trusted source
Deep-sea pressure found to preserve food for microbes in the abyss
by Birgitte Svennevig, University of Southern Denmark
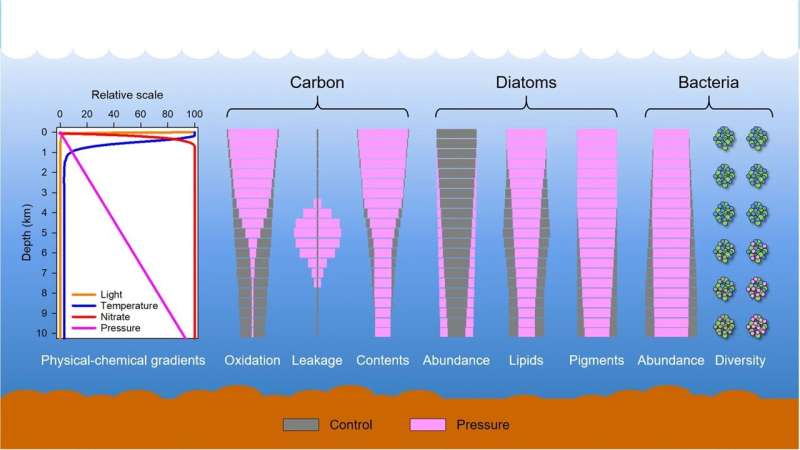
A new study from the Danish Center for Hadal Research reports on a series of experiments with exposing marine snow to increasing pressure—up to 1000 bar, which corresponds to the pressure at the bottom of some of the world's deep-sea trenches, 10 km below the sea surface.
Marine snow is millimeter-sized flakes, created when sticky, dead cells at the sea surface clump together with other dead or dying cells, particles, and bacteria and sink to the bottom. The organic material can be dead algae, dead small animals, or their feces. Together, it is called marine snow because the flakes look like snow as they sink through the water column . There can be hundreds of different bacteria in one flake in addition to particles of organic matter .
"Not much is known about how marine snow responds to the increasing pressure when it sinks. However, it is known that marine snow is food for many microbes and small animals on the seabed. In fact, there are more microbes in the part of the ocean that lies at or below 1000 meters depth than anywhere else on Earth," says biologist Peter Stief, the lead author of the study.
"This habitat is extremely large, and there can be a long distance between the microbes down there, but nevertheless, a huge number of Earth's organisms thrive under high pressure , and we don't know how,"
Other authors are Ronnie N. Glud, Clemens Schauberger, Kevin W. Becker, Marcus Elvert, John Paul Balmonte, Belén Franco-Cisterna and Mathias Middelboe. The study is published in the journal Communications Earth & Environment .
In addition to the marine snow bringing nutrients such as organic carbon , nitrogen, sulfur, and phosphate down to the microbes in the deep, it also contributes to the burial of huge amounts of carbon in the seabed. This happens when an alga during its lifetime absorbs CO 2 from the atmosphere, dies, and sinks to the bottom, taking the carbon with it. Most marine snow is eaten by animals or broken down by microbes, but a small part is stored on the sea floor.
"Perhaps only 1% of the marine snow gets stored on the seabed. But over time, it accumulates to huge amounts. The oil and gas we are currently extracting is largely created in this way," says Peter Stief.
Organic matter also reaches the deep-sea microbes in other ways. Data from a number of deep-sea expeditions carried out by the Danish Center for Hadal Research have shown that large sediment slides can occur on slopes in the deep sea. Such sediment slides can instantly send large amounts of nutrient-rich sediment down into the deepest trenches.
Now, Peter Stief and colleagues show that another mechanism also ensures that organic matter can reach the deep.
In the basement under University of Southern Denmark, the hadal researchers have pressure tanks for experiments. The tanks can withstand 1000 bar pressure, corresponding to the pressure at a depth of 10 km.
The team filled five pressure tanks with seawater and flakes of marine snow that they had made from diatoms and bacteria. Each flake was approx. 2 mm in diameter. By letting the pressure tanks rotate horizontally all the time, the researchers ensured that the flakes remained sinking in water without reaching the bottom of the tank, thus simulating the constant sinking of the flakes. Every day, they increased the pressure in the tanks by 50 bar to simulate the pressure at ever-increasing depths.
After four days, the flakes were under 200 bar pressure, corresponding to an ocean depth of 2 km. Then, the researchers opened the first tank to see how the flakes did. The same is true after 400 bar pressure, 600 bar pressure, 800 bar pressure, and the last one after 1000 bar pressure.
"We could see that the bacteria's respiration decreased as pressure increased; this means that they 'ate' less organic carbon. At 600 bar, respiration stopped completely and remained so all the way down to 1000 bar pressure, where approx. half of a flake remained—and thus food for the pressure-adapted seabed microbes," says Peter Stief.
Journal information: Communications Earth & Environment
Provided by University of Southern Denmark
Explore further
Feedback to editors

Quality control: Neatly arranging crystal growth to make fine thin films
3 minutes ago

Pilot study uses recycled glass to grow plants for salsa ingredients
4 hours ago

Study shows continuous manufacturing reduces cultivated meat costs

Chalk-based coating creates a cooling fabric

Researchers teach artificial intelligence about frustration in protein folding
17 hours ago

New view of North Star reveals spotted surface
18 hours ago

Trees stripped by invasive caterpillars muster defenses that can harm native insects, research shows
20 hours ago

Habitat connectivity drives panda recovery, finds study

Advanced materials could provide more durable metals for fusion power reactors
21 hours ago

Paleontologists describe new examples of giant sea scorpions from the Silurian and Devonian in New South Wales
Relevant physicsforums posts, homo naledi: 5 yr update & new findings (2021), hiking illness danger -- rhabdomyolysis.
Aug 18, 2024
Toxic Chemicals Found on old books
Strategies and tips for first responders interacting with autism spectrum disorder patients.
Aug 16, 2024
Cannot find a comfortable side-sleeping position
Using capsaicin to get really high.
More from Biology and Medical
Related Stories

Disproportionately large amounts of carbon accumulate at the bottom of deep-sea trenches, research shows
Dec 21, 2022

Team investigates consequences of Deepwater Horizon oil spill
Jun 11, 2019

A glimpse into the ocean's biological carbon pump
Sep 23, 2021

Researchers find long-banned pollutants in the very deepest part of the ocean
May 1, 2023

Environmental toxin PCB found in deep sea trench
Apr 17, 2023

A globally important microbial process hidden on marine particles
Jul 2, 2021
Recommended for you

New fish species discovered in Mauritanian deep-water coral reefs
22 hours ago

Sponges' symbiosis with bacteria helps them store toxic molybdenum to keep predators away, study shows

'Amazon' algae shed light on what happens to populations when females switch to asexual reproduction
23 hours ago

First spatial map of malaria infection in the liver opens new possibilities for more effective treatments
Let us know if there is a problem with our content.
Use this form if you have come across a typo, inaccuracy or would like to send an edit request for the content on this page. For general inquiries, please use our contact form . For general feedback, use the public comments section below (please adhere to guidelines ).
Please select the most appropriate category to facilitate processing of your request
Thank you for taking time to provide your feedback to the editors.
Your feedback is important to us. However, we do not guarantee individual replies due to the high volume of messages.
E-mail the story
Your email address is used only to let the recipient know who sent the email. Neither your address nor the recipient's address will be used for any other purpose. The information you enter will appear in your e-mail message and is not retained by Phys.org in any form.
Newsletter sign up
Get weekly and/or daily updates delivered to your inbox. You can unsubscribe at any time and we'll never share your details to third parties.
More information Privacy policy
Donate and enjoy an ad-free experience
We keep our content available to everyone. Consider supporting Science X's mission by getting a premium account.
E-mail newsletter
Advanced science. Applied technology.

Deep Ocean Pressure Simulation Testing
- Energy & Environment
- Oil & Gas
- Subsea Engineering & Offshore Technology
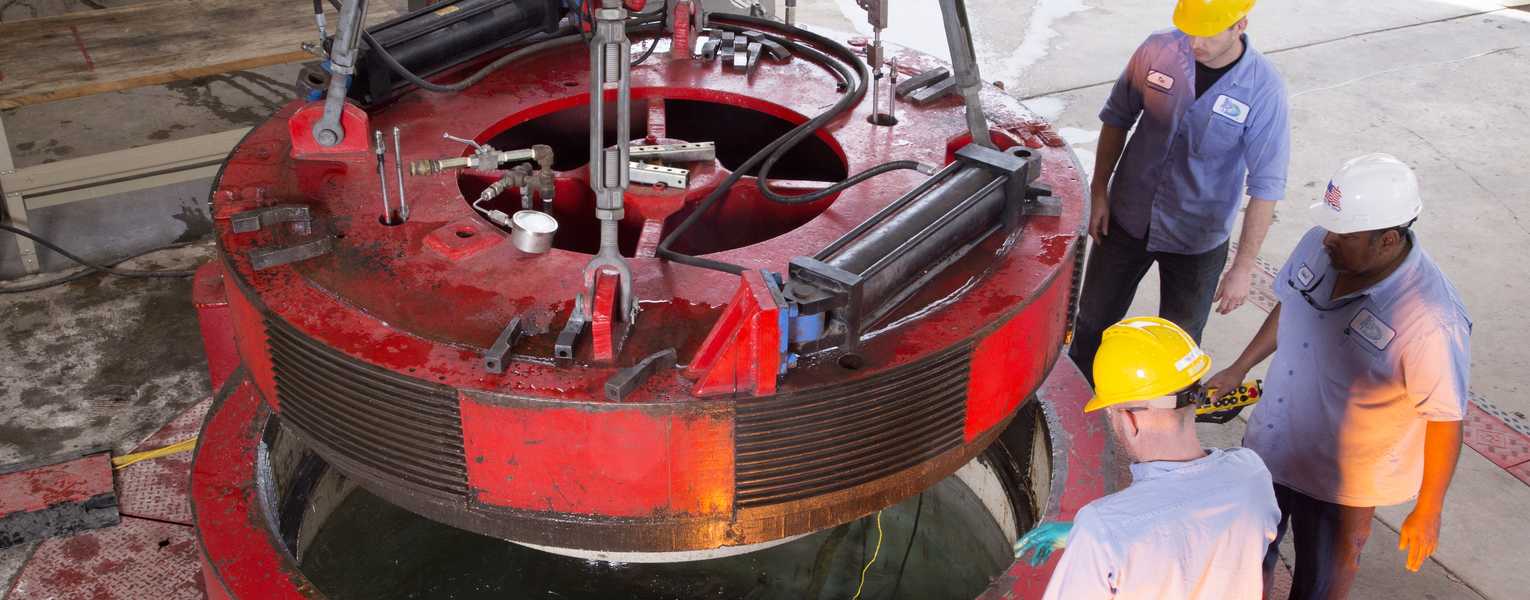
With more than 50 years of experience in offshore and marine technologies, Southwest Research Institute (SwRI) offers a wide variety of services to meet the need for deep ocean pressure simulation testing. These pressure simulation services provide a final check of quality and operational integrity for clients including:
- Oil producers
- Manufacturers of subsea components
- Pipeline manufacturers
Pressure Simulation Services
- Structural analysis and experimental strain/stress measurement technology
- Deep ocean pressure simulation testing
- Test services for offshore insulation and corrosion protection systems
- Performance verification testing of valves and components
- Standard tests for offshore equipment
Pressure Simulation Facilities
Our Ocean Engineering and Structural Testing Laboratory in San Antonio, Texas, has more than 10,500 square feet of climate-controlled laboratory space with additional outdoor test facilities. Deep-ocean pressure simulation test chambers range from 90 inches inside diameter and 20 feet deep, to 16 inches inside diameter and 30,000 psig, and are used for:
- Static testing
- Cycle testing
- Destruction testing
- Internal and external hydrostatic pressure tests
- Stress analysis and acceptance tests
- Operational tests requiring electrical and hydraulic penetrations
- API steel pipe casing
- Fiberglass pipe
- Stainless steel pipe
- Prototype equipment
- Pressure housings
- Subsea instrumentation
- Oilfield production equipment
For more information about deep ocean pressure simulation testing, please contact Joe Crouch at +1 210 522 4295 or Chris Storey at +1 210 522 3992.
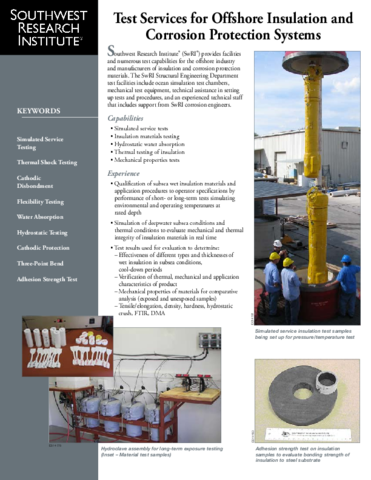
- Bienvenidos
Information
- Author Services
Initiatives
You are accessing a machine-readable page. In order to be human-readable, please install an RSS reader.
All articles published by MDPI are made immediately available worldwide under an open access license. No special permission is required to reuse all or part of the article published by MDPI, including figures and tables. For articles published under an open access Creative Common CC BY license, any part of the article may be reused without permission provided that the original article is clearly cited. For more information, please refer to https://www.mdpi.com/openaccess .
Feature papers represent the most advanced research with significant potential for high impact in the field. A Feature Paper should be a substantial original Article that involves several techniques or approaches, provides an outlook for future research directions and describes possible research applications.
Feature papers are submitted upon individual invitation or recommendation by the scientific editors and must receive positive feedback from the reviewers.
Editor’s Choice articles are based on recommendations by the scientific editors of MDPI journals from around the world. Editors select a small number of articles recently published in the journal that they believe will be particularly interesting to readers, or important in the respective research area. The aim is to provide a snapshot of some of the most exciting work published in the various research areas of the journal.
Original Submission Date Received: .
- Active Journals
- Find a Journal
- Proceedings Series
- For Authors
- For Reviewers
- For Editors
- For Librarians
- For Publishers
- For Societies
- For Conference Organizers
- Open Access Policy
- Institutional Open Access Program
- Special Issues Guidelines
- Editorial Process
- Research and Publication Ethics
- Article Processing Charges
- Testimonials
- Preprints.org
- SciProfiles
- Encyclopedia
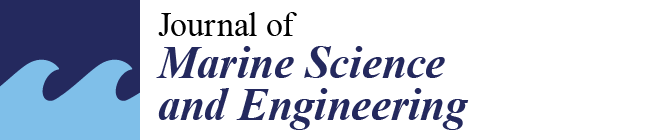
Article Menu
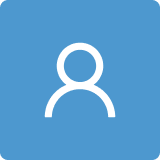
- Subscribe SciFeed
- Recommended Articles
- Google Scholar
- on Google Scholar
- Table of Contents
Find support for a specific problem in the support section of our website.
Please let us know what you think of our products and services.
Visit our dedicated information section to learn more about MDPI.
JSmol Viewer
An experimental study of pulp-lift characteristics using a high-viscous fluid simulating deep muddy seawater.

1. Introduction
2. materials, 2.1. working fluid, 2.2. viscosity experiments, 2.3. results, 2.4. estimation of the viscosity curve, 3. pulp-lift experiments, 3.1. experimental conditions, 3.1.2. velocity conditions, 3.1.3. observation conditions, 3.2. results, 3.2.1. pulp-lift results, 3.2.2. reynolds number, 3.2.3. pulsation, 4. discussion, 4.1. results of lifting ore, 4.2. effects of pulsation caused by increased viscosity, 5. conclusions, author contributions, institutional review board statement, informed consent statement, data availability statement, acknowledgments, conflicts of interest.
- Kato, Y.; Fujinaga, K.; Nakamura, K.; Takaya, Y.; Kitamura, K.; Ohta, J.; Toda, R.; Nakashima, T.; Iwamori, H. Deep-Sea Mud in the Pacific Ocean as a Potential Resource for Rare-Earth Elements. Nat. Geosci. 2011 , 4 , 535–539. [ Google Scholar ] [ CrossRef ]
- Bernard, J.; Bath, A.; Greger, B. Analysis and Comparison of Nodule Hydraulic Transport Systems. In Proceedings of the Offshore Technology Conference, Huston, TX, USA, 27–30 April 1987. [ Google Scholar ]
- Yamazaki, T.; Nakatani, N.; Arai, R.; Sekimoto, T.; Katayama, H. Combined Mining and Pulp-Lifting of Ferromanganese Nodules and Rare-Earth Element-Rich Mud around Minamitorishima Island in the Western North Pacific: A Prefeasibility Study. Minerals 2021 , 11 , 310. [ Google Scholar ] [ CrossRef ]
- Sugahara, H.; Shimomura, K.; Nakatani, N.; Yamazaki, T.; Katayama, H.; Kumagai, T. Fundamental Study of Pulp Lift Method for Mixed Lifting of Submarine Mineral Resources. Jpn. J. JSCE 2023 , 79 , 23–18091. [ Google Scholar ] [ CrossRef ]
- Orita, K.; Tani, K.; Suzuki, A.; Kosho, T. Study of Ore Lifting Efficiency Using Mixture of Bentonite Suspension and Sand for Deep Sea Mining. J. JSCE Ser. B3 2021 , 77 , I_523–I_528. [ Google Scholar ] [ CrossRef ]
- Nunoya, N.; Tsuchida, T.; Abe, T. Flow Characteristics of Marine Clays of High Water Content Condition. J. Jpn. Soc. Civ. Eng. Ser. B2 (Coast. Eng.) 2012 , 68 , I_551–I_555. [ Google Scholar ] [ CrossRef ]
- Japanese Standards Association (1991). JIS Z 8803: Methods for Viscosity Measurement of Liquid. 2011. Available online: https://kikakurui.com/z8/Z8803-2011-01.html (accessed on 19 July 2024).
- Shimizu, Y.; Hatakeyama, N.; Hanamura, E.; Watanabe, K.; Yokoyama, Y.; Masuyama, T. Flow Characteristics of Slurry with Rare-Earth Rich Mud under Deep Seabed around Minamitorishima. J. Min. Mater. Process. Inst. Jpn. 2019 , 135 , 52–62. [ Google Scholar ] [ CrossRef ]
- Asai, K.; Ichiyanagi, M.; Satone, H.; Mori, T.; Tsubaki, J.; Itoh, Y. The Influence of Non-Newtonian Property on the Apparent Viscosity Measured by Single Cylinder Rotational Viscometer (Type-B Viscometer). J. Soc. Powder Technol. Jpn. 2009 , 46 , 873–880. [ Google Scholar ] [ CrossRef ]
- Hatakeyama, N.; Shimizu, Y.; Masuyama, T. Spreadsheet Solutions for Rheological Properties and Viscoplastic Fluid Flow in Circular Pipes and in Parallel-Plates. J. Min. Mater. Process. Inst. Jpn. 2019 , 135 , 15–24. [ Google Scholar ] [ CrossRef ]
- Orita, K.; Tani, K.; Kosho, T.; Suzuki, A.; Tanaka, K. Study of Drag Force to Falling Spheres in Carrier Materials (Mixture of Viscous Fluid and Fine Particles) for Ore Lifting Technology and Interpretation of Rheological Constants. J. JSCE Ser. B3 2020 , 76 , I_881–I_886. [ Google Scholar ] [ CrossRef ]
Click here to enlarge figure
Working Fluid | Density [kg/m ] |
---|---|
Water | 999.9 |
CMC 0.5% | 1031 |
CMC 0.75% | 1007 |
CMC 1.0% | 1015 |
CMC 1.2% | 1090 |
Ore | Diameter [mm] | Mass [g] | Density [kg/m ] |
---|---|---|---|
Aluminum small (A10) | 10 | 1.47 | 2800 |
Aluminum medium (A20) | 20 | 11.7 | 2800 |
Aluminum large (A30) | 30 | 39.7 | 2810 |
Manganese Nodules | 30 | 7.07 | 2000 |
Title | Contents |
---|---|
Working fluid | Water, CMC 0.5%, CMC 0.75%, CMC 1.0%, CMC 1.2% |
Velocity [m/s] | 0.5, 1.0, 1.5 |
Ore | A10, A20, A30 |
Height [m] | 5.0 |
No. | V [m/s] | Ore | Working Fluid | |||
---|---|---|---|---|---|---|
Water | CMC 0.5% | CMC 1.0% | CMC 1.2% | |||
0.5 | A10 | - | 〇 | 〇 | 〇 | |
A20 | - | 〇 | 〇 | 〇 | ||
A30 | - | 〇 | 〇 | 〇 | ||
1.0 | A10 | 〇 | 〇 | 〇 | 〇 | |
A20 | - | 〇 | 〇 | 〇 | ||
A30 | - | 〇 | 〇 | 〇 | ||
1.5 | A10 | 〇 | 〇 | 〇 | 〇 | |
A20 | 〇 | 〇 | 〇 | - | ||
A30 | 〇 | 〇 | 〇 | - |
The statements, opinions and data contained in all publications are solely those of the individual author(s) and contributor(s) and not of MDPI and/or the editor(s). MDPI and/or the editor(s) disclaim responsibility for any injury to people or property resulting from any ideas, methods, instructions or products referred to in the content. |
Share and Cite
Onishi, S.; Itagaki, Y.; Nakatani, N.; Ohara, K.; Katayama, H.; Yamazaki, T. An Experimental Study of Pulp-Lift Characteristics Using a High-Viscous Fluid Simulating Deep Muddy Seawater. J. Mar. Sci. Eng. 2024 , 12 , 1448. https://doi.org/10.3390/jmse12081448
Onishi S, Itagaki Y, Nakatani N, Ohara K, Katayama H, Yamazaki T. An Experimental Study of Pulp-Lift Characteristics Using a High-Viscous Fluid Simulating Deep Muddy Seawater. Journal of Marine Science and Engineering . 2024; 12(8):1448. https://doi.org/10.3390/jmse12081448
Onishi, Sakai, Yurie Itagaki, Naoki Nakatani, Kyara Ohara, Hiroyuki Katayama, and Tetsuo Yamazaki. 2024. "An Experimental Study of Pulp-Lift Characteristics Using a High-Viscous Fluid Simulating Deep Muddy Seawater" Journal of Marine Science and Engineering 12, no. 8: 1448. https://doi.org/10.3390/jmse12081448
Article Metrics
Further information, mdpi initiatives, follow mdpi.
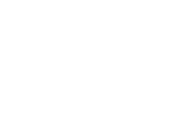
Subscribe to receive issue release notifications and newsletters from MDPI journals

An official website of the United States government
The .gov means it’s official. Federal government websites often end in .gov or .mil. Before sharing sensitive information, make sure you’re on a federal government site.
The site is secure. The https:// ensures that you are connecting to the official website and that any information you provide is encrypted and transmitted securely.
- Publications
- Account settings
Preview improvements coming to the PMC website in October 2024. Learn More or Try it out now .
- Advanced Search
- Journal List
- Sensors (Basel)
- PMC11314998

A Rapid Nanofocusing Method for a Deep-Sea Gene Sequencing Microscope Based on Critical Illumination
1 Changchun Institute of Optics, Fine Mechanics and Physics, Chinese Academy of Sciences, Changchun 130033, China
2 University of Chinese Academy of Sciences, Beijing 100049, China
3 State Key Laboratory of Applied Optics, Changchun 130033, China
4 Key Laboratory of Optical System Advanced Manufacturing Technology, Chinese Academy of Sciences, Changchun 130033, China
Fengfeng Shu
Wenchao zhou, shixun zhao, associated data.
The original contributions presented in the study are included in the article/ Supplementary Materials . Further inquiries can be directed to the corresponding authors.
In the deep-sea environment, the volume available for an in-situ gene sequencer is severely limited. In addition, optical imaging systems are subject to real-time, large-scale defocusing problems caused by ambient temperature fluctuations and vibrational perturbations. To address these challenges, we propose an edge detection algorithm for defocused images based on grayscale gradients and establish a defocus state detection model with nanometer resolution capabilities by relying on the inherent critical illumination light field. The model has been applied to a prototype deep-sea gene sequencing microscope with a 20× objective. It has demonstrated the ability to focus within a dynamic range of ±40 μm with an accuracy of 200 nm by a single iteration within 160 ms. By increasing the number of iterations and exposures, the focusing accuracy can be refined to 78 nm within a dynamic range of ±100 μm within 1.2 s. Notably, unlike conventional photoelectric hill-climbing, this method requires no additional hardware and meets the wide dynamic range, speed, and high-accuracy autofocusing requirements of deep-sea gene sequencing in a compact form factor.
1. Introduction
Approximately three-quarters of Earth’s surface is covered by oceans, with half being deep-sea regions where the depth surpasses 1000 m [ 1 ]. These zones are characterized by the absence of light and the presence of water temperatures that are close to freezing. However, they also exhibit the presence of hydrothermal vents that erupt at temperatures that exceed 400 °C [ 2 , 3 ]. The deep-sea environment is characterized by extreme pressure, with the Mariana Trench representing a depth of 1100 atmospheres. Salinity levels are also elevated in this milieu [ 4 ]. Microbes that thrive in these extreme conditions, known as extremophiles, possess genetic sequences that are not yet fully understood by scientists. These sequences enable the microbes to produce enzymes resilient to diverse temperatures, salinity levels, pH, and pressures [ 5 , 6 ]. The discovery of these genetic sequences is expected to have a significant impact on various sectors, including agriculture, pharmaceutical manufacturing, and the field of bioengineering [ 7 , 8 ].
Despite the significant progress that has been made in the field of deep-sea microbial genetics, the majority of analyses are conducted on land following the extraction of samples from the deep sea [ 9 , 10 ]. The transition in the environment has the unintended consequence of introducing deviation into the results [ 11 , 12 ]. This is particularly problematic in the context of deep-sea genetic sequencing, where the lack of specialized equipment represents a significant challenge [ 13 , 14 ]. In contrast to laboratory-based sequencers, which operate in stable temperatures and humidity, those deployed in the deep sea must endure isolated, extremely harsh conditions over long periods. These instruments are limited in space by the compression of the cabin and must withstand severe shock during descent. They operate fully automatically on the sea floor for months or even a year. The temperature inside the cabin is in the range of 0 °C to 20 °C. During that time, they cannot be maintained [ 15 , 16 , 17 ]. There is a critical need to develop autofocusing mechanisms that are highly integrated, resilient, and possess a wide detection range to ensure the successful operation of in situ deep-sea gene sequencing equipment.
Conventional sequencers employ photoelectric sense systems to localize the focal plane [ 18 , 19 , 20 , 21 ]. Zhang proposes two laboratory autofocusing methods based on multi-position laser differential confocal and laser-based arrayed spots, respectively. The former has a focusing accuracy of 1/10 of the depth of focus, and the latter has a dynamic range of ±100 μm, but not both [ 22 , 23 ]. However, these approaches are often structurally complex and offer inadequate dynamic ranges. In such systems, light emitted from a dedicated source is reflected off the imaging surface and detected, enabling the determination of the out-of-focus surface’s position. This information is then used by an actuator to correct any defocus. While these techniques share an optical path with the imaging system, either entirely or in part, substantial temperature shifts and severe impacts may cause the systems to lose cofocus. It would be very challenging to recalibrate in a deep-sea environment [ 24 , 25 , 26 , 27 , 28 ].
This study introduces a focus detection method that identifies the edges of the critical illumination field under various defocus conditions and is seamlessly integrated with the gene sequencing imaging system. Differing from traditional, edge detection approaches, our method pins down the gradient extremities to discern the defocus by measuring the position shift between the field’s opposing edges. This difference serves as the metric for evaluating defocus. The system employs its built-in detector to capture the differences in the illumination field’s size across varying degrees of defocus. These differences are then used to generate a standard evaluation curve, which correlates the defocus amount with the metric. This curve is used to determine the autofocus mechanism precisely. Experiments on the deep-sea genetic sequencing autofocus microscope prototype substantiated the approach’s efficacy. The results indicate that within a dynamic range of ±40 μm, the accuracy of detection of a single defocused image can reach 200 nm. Moreover, the accuracy can be raised to 78 nm by accumulating multiple images through continuous sampling. Additional iterations can broaden the dynamic range beyond ±100 μm after two cycles. This robust method, which is impervious to perturbations such as laser energy variation and displacement, represents a viable autofocusing solution for deep-sea, in situ genetic sequencing.
The optical apparatus in the epi-illumination fluorescence microscope is designed to operate using two techniques: Köhler illumination and critical illumination [ 29 , 30 , 31 ]. In the field of genetic sequencing, critical illumination is preferred due to its superior ability to modulate the shape, depth, intensity, consistency, and homogeneity of the excitation light field [ 32 , 33 , 34 ]. Genetic sequencing scanners employ square critical illumination light fields, thereby enhancing the utilization factor of the sequencing chips. Concurrently, excitation light reflected off sequencing chips is typically regarded as noise and is consequently filtered out. In this study, we exploit these extraneous light fields, utilizing them as a focus detecting optical signal within the system’s autofocus feature.
Figure 1 illustrates the optical configuration of a multichannel fluorescence microscope optimized for genetic sequencing. The system comprises an infinity-corrected objective tube lens, and a camera, collectively establishing an imaging mechanism. The laser illumination part of the prototype imaging system consists of a collimating lens, a laser, a square multimode fiber, and a diffuser. The diffuser facilitates beam homogenization. Laser illumination, after transmission through the multimode fiber and collimation into a parallel beam, is focused by the microscope objective onto the genetic sequencing chip at the focal plane. The gene sequencing chip consists of a silicon substrate and a glass cover, with channels spaced 170 μm apart to facilitate the growth of DNA clusters on the silicon surface. The silicon’s surface serves as a reflective mirror, redirecting the excitation light back into the objective lens. To discern fluorescence signals within specific spectral ranges while excluding extraneous light from reflected laser beams, the microscope’s detection system employs a filter wheel. In the empty channel, this wheel permits reflected laser light to strike the detector’s surface directly, generating a focused detection signal.

Critical illumination fluorescence microscopy imaging system. CO is a collimator. MF is a multimode optical fiber with a square output section. De-Speckler is a speckle-beam homogenizer.
Figure 2 a depicts the optical imaging pathway of the genetic sequencing microscope, which includes both an objective lens and a tube lens. The critical illumination system directs the light source onto the focal plane of the objective. In the absence of a cutoff filter, the conjugate image materializes on the focal plane of the eyepiece, as shown in Figure 2 b. The diagram above Figure 2 c displays the one-dimensional, grayscale intensity profile of the conjugate image’s square critical illumination light field outlined in Figure 2 b. As the distance of the object alters, adjusting the image distance is necessary to preserve optimal imaging. While sharp images are obtainable despite alterations in the object and image distance, concurrent changes in magnification are inevitable. The amount of out-of-focus z is defined as 0 at the focal plane of the lens, with negative values closer to the lens and positive values further from the lens. In real-world applications, due to the invariable image distance, defocus arises. Figure 2 c is a diagram of the location of the lines shown in Figure 2 b. Figure 2 c portrays the actual one-dimensional grayscale profile of the defocused light field, and the lower diagram depicts its first-order derivative or gradient profile. Defocusing results in blurring of the boundaries of the light field, yet the gradient extremes remain. The separation between these extremes depends on both the magnification and the state of defocus of the system. As indicated in Figure 2 c, the optimal focus is attained when the separation d z is zero. Defocusing alters the magnitude and position of the edge gradients due to edge blurring and changes in magnification.

The principle of critical illumination focuses on feedback. ( a ) The optical path for the infinite-range microscope. The red and green lines represent the propagation path of light rays at the edge of the field of view. ( b ) Simulation of square laser illumination output end-face field distribution. ( c ) Intensity and gradient simulation distribution at the line position in ( b ). ( d ) The gradient distributions T 1 , T 2 and the positional difference of the gradient extrema D 1 , D 2 before and after the displacement of the light field.
In light of these observations, we introduce an innovative autofocus approach for genetic sequencing, leveraging the critical illumination light field as an optical feedback signal. This strategy not only streamlines the complexity of the system and shrinks its spatial footprint but is also unaffected by displacement of the light field. Figure 2 d depicts the concept behind this interference immunity. The deployment and recovery process of deep-sea gene sequencers is accompanied by strong shocks that cause changes in the position of the light field. Since the excitation light for the sequencer comes from compact, rigid laser emission surfaces such as optical fibers or light homogenizing rods, the polar position difference D 1 is equal to D 2 .
3.1. Simulation Analysis
The calculation of image gradients represents a pivotal methodology for the identification of edge information in visual data. This process quantifies the change in image sizes, whereby regions exhibiting edges exhibit elevated gradient magnitudes due to marked variations in intensity. Edge classes are predominantly comprised of step, ridge, slope, and pulse variations [ 35 , 36 ]. The different categories are rooted in the speed of intensity change. The edge of the critically illuminated field is the ridge shape with the fastest intensity change. Figure 2 c displays both theoretical and practical profiles of ridge-type edge fluctuation, in addition to the gradient estimation curve derived from first-order derivative edge detection operators. The process of gradient computation involves the calculation of derivatives. Nevertheless, given the discrete nature of image matrices, such changes are estimated via differential approximations. The approximate values of gradients—essentially estimated derivatives—are determined by gauging the variation in pixel intensity [ 37 , 38 ]. For any given pixel with luminance g x , y , its gradient magnitude can be expressed as follows:
Gradient (difference) in the x-direction:
To validate our analysis, critical illumination microscopy was performed using ZEMAX2013 to obtain conjugate images of defocused critical illumination light fields at different defocus positions, as shown in Figure 3 a. The simulation was performed using a 30x infinity microscope objective model with f = 8 mm, a laser collimator with f = 12 mm, and an air-spaced achromatic doublet with f = 200 mm as a converging tube lens. The light source size is 1 × 1 mm; the detector size is set to 20 × 20 mm with 4000 × 4000 pixels. The intensity distribution along the x -axis at the same location, as shown in Figure 3 a, is presented in Figure 3 b. Figure 3 c illustrates the application of a first-order derivative edge operator to analyze the gradient in the simulated conjugate images for differing defocus states. The results indicate a notable decline in the magnitude of edge gradient extremes (maxima and minima) and a corresponding shift in their positions as defocus intensity is increased. A robust correlation was discerned between the magnitude of edge gradients, their positions, and defocus levels.

Simulation of the defocused critical illumination light field. ( a ) Conjugate images of the excitation field at five defocused positions. ( b ) One−dimensional intensity curves in the x-direction of the conjugate images of the excitation field at five defocused positions. ( c ) One−dimensional gradient curves in the x−cut direction of the conjugate images of the excitation field at five defocused positions.
This paper describes an optoelectronic autofocus method. This type obtains the defocus evaluation value by processing and calculating the detected optical or electrical signals to obtain the defocus evaluation value. The method in this paper detects the one-dimensional energy distribution of the optical field under critical illumination conditions and calculates the positional difference between the maximum and minimum values of the gradient of the energy distribution as the differential defocus evaluation value. This value is essentially a distance or width metric. Later we will use “t” to represent the defocus evaluation value, for example, the vertical coordinate in Figure 4 d. Before the first autofocus is performed, a series of equally spaced statistics corresponding to a known defocus evaluation value is required, and a standard defocus evaluation curve is constructed by fitting these data. This curve shows the direct relationship between the defocus distance and the defocus evaluation value. Figure 4 a shows two normalized standard defocus evaluation curves. In the autofocus process, the current defocus evaluation value is first obtained by detection and calculation, and then the standard evaluation curve is used to determine the degree of defocus, which is finally compensated by the actuator.

Simulation of standard evaluation curves for defocus distances. ( a ) The black line is the normalized standard defocus distance evaluation curve, plotted using the gradient extremes as the defocus evaluation value. The red line is the normalized standard defocus distance evaluation curve, plotted using the gradient extremum position difference as the defocus evaluation value. ( b ) The black line is the normalized standard defocus distance evaluation curve, plotted using the second derivative extremum as the defocus evaluation value. The red line is the normalized standard defocus distance evaluation curve, plotted using the second derivative extremum position difference as the defocus evaluation value. ( c ) The theoretical magnification of the system under defocusing and the actual magnification obtained from edge recognition. ( d ) Standard evaluation curves for different objective lens focal lengths. ( e ) Standard evaluation curves for different illumination widths.
Standard evaluation curves were individually plotted to demonstrate these observed patterns, as shown in Figure 4 a. The evaluation curve correlating with gradient extremes displays a near-quadratic relationship, peaking around the focal plane. This results in a drastic reduction in focus sensitivity within its vicinity, which impedes the ability to discern defocus direction from a solitary assessment and adversely affects the accuracy and efficiency of autofocus. The focus sensitivity is quantified as the slope of the evaluation curve. Conversely, the evaluation curve associated with positional differences of gradient peaks closely resembles a linear relationship, suggesting consistent focus sensitivity across the entire dynamic range. This enables enhanced one-shot autofocus accuracy, as the linearity of the curve allows for a more accurate estimation of focus. We also performed a simulation analysis using grayscale, second-order derivative poles and their positions. The higher order derivative analysis exhibits higher sensitivity, as evidenced by the faster rate of change of the second-order derivative extremes than the first-order derivative extremes in Figure 4 b. However, the dynamic range is significantly reduced and more susceptible to noise, resulting in a significant increase in bias. The second-order grayscale derivatives at the edges of both sides of the optical field also show extremes with the same position difference as the first-order derivatives. It can be seen that the sensitivity of the focusing method based on extreme position differences is not improved by the use of higher-order derivative analysis but can lead to a larger deviation.
In optimal imaging conditions, variations in object distance prompt corresponding adjustments in image distance, allowing for an exact calculation of system magnification via Newton’s formula. However, in cases where image distance does not vary with object distance, system magnification calculation fails when the system is defocused. In such instances, the edge is usually the location of the gradient extremes. Consequently, by measuring the inter-edge distance to determine the image size and comparing it with the object size, we can derive the system magnification at that particular focus. Figure 4 c illustrates the relationship between magnification under such circumstances and the deviation from the intended focal plane. It demonstrates an increase in the discrepancy from ideal magnification with enhanced defocus distance and a consequent diminished change in magnification tending towards linearity.
The slope of the standard evaluation curve is indicative of focus sensitivity. We conducted a thorough analysis of the various elements that influence this sensitivity. The positional disparity of the peak gradient value at zero defocus is designated as d 0 , and the defocus evaluation metric t represents the difference between the positional deviation d of the peak gradient value at defocus z and d 0 . We created standard evaluation curves of t against defocus z. As illustrated in Figure 4 d, these curves are derived from sampling at 5 μm intervals within a ±100 μm defocus range and utilizing objectives with various focal lengths. It indicates that objectives with shorter focal lengths exhibit a higher absolute value of the slope in the standard evaluation function. This is attributable to their larger magnification changes in response to equivalent object distance alterations, thereby enhancing focus sensitivity.
The grayscale data captured by the detector is discrete, which means that the smallest discernible change in the position of gradient extrema is one pixel. This one-pixel variation corresponds to the smallest detectable defocus amount, which defines the system’s detection limit (peak sensitivity). To enhance sensitivity, it is possible to reduce the pixel size and increase the quantity, or alternatively, to expand the size of the critical illumination excitation light field in order to cover more pixels under the same magnification change conditions, as illustrated in Figure 4 e.
3.2. Accuracy Test
The prototype of the multichannel fluorescence microscope for gene sequencing constructed according to Figure 1 is shown in Figure 5 . The functional area of a gene sequencing chip is much larger than the imaging field of view, so a two-dimensional moving platform must be used to scan all the DNA clusters. After moving to each new location, the system first determines the focal plane. Once the illumination laser is turned on, the light is transmitted through an optical fiber to a collimator and into the microscope, where a dichroic beam splitter then reflects the laser light into the objective lens. The collimator and objective form a critical illumination system that projects the light field from the square fiber port onto the chip surface. The laser light field is reflected from the chip surface, and the objective collects this light and directs it into the system. A portion of the intense laser light passes through a dichroic beam splitter and is imaged onto the target surface of the detector. The system then collects an image of the light field and extracts the difference in position of the gradient peaks of the image to generate a standardized evaluation curve and calculate the current amount of defocus, which is then compensated by a z -axis displacement actuator. The focusing process is fully automated with the detector taking 40 ms to acquire an image, the actuator compensating the defocus amount in less than 100 ms, and the control program and algorithm calculating the amount in about 20 ms. The total time for a single focusing iteration is less than 160 ms. After focusing, the system turns the illumination laser back on and rotates the filter wheel to obtain DNA cluster site images in four different spectral bands. The system turns the illumination laser back on and rotates the filter wheel to obtain images of the DNA clusters in four different spectral bands.

Prototype of a multichannel fluorescence microscope for gene sequencing. IPC: Industrial Personal Computer. Camera: Andor Zyla 4.2, Andor Technology, Belfast, UK. Illumination: semiconductor laser (Changchun New Industries, MDL-E-655, Changchun, China). Tube lens: Thorlabs TTL100-A, Thorlabs Inc., Newton, NJ, USA. Filter wheel: Thorlabs FW102C. Optical fiber: Changchun New Industries multimode optical fiber. Beamsplitter: Chroma ZT532/660rpc, MEETOPTICS, Barcelona, Spain. Microobjective: Olympus UCPLFLN20X (Olympus, Tokyo, Japan) with a numerical aperture of 0.7 and a magnification of 20×. Motion module: A one-dimensional linear displacement mechanism (WDI, ZAA-STD) was selected to provide a travel range of 10 cm with a minimum step displacement of 78 nm. The laser enters the color from right to left in the direction of the arrow and then shines downward. Reflected light propagates from bottom to top, with some continuing to propagate upward through the dichroic film.
In our experiments, we stabilized the sequencing chip and modulated the objective to acquire conjugate images of the excitation field across various defocus stages. Images were captured at 1 μm intervals within a defocus range of ±100 μm, resulting in the acquisition of 200 images of the defocused excitation field. As illustrated in Figure 6 a, images exhibiting defocus discrepancies of 25 μm were selected. It can be observed that the excitation field’s dimensions undergo a progressive reduction as transitions from negative to positive defocus values occur. Our previous simulation studies have indicated that reducing the pixel size or enlarging the excitation field’s area increases the number of pixels reacting to magnification changes, thereby enhancing sensitivity. To this end, we applied interpolation to the acquired defocused images. Figure 6 b displays 40 images selected at 5 μm intervals from the original 200, which were employed in fitting a standard curve. This yielded an R 2 value of 0.99619.

Extraction of standard evaluation curves for defocusing amounts. ( a ) Image of the excitation field of an equidistant defocused volume captured by the detector. ( b ) Standard evaluation curve of defocusing amount plotted at 5 μm intervals. ( c ) Standard evaluation curve of defocusing amount plotted at 1 μm intervals.
The experimental data exhibited exceptional linearity and minimal variance within a ±50 μm defocus range. Based on this, 100 images were selected at 1 μm intervals within a ±50 μm range to obtain the standard evaluation function for the defocus detection system, as shown in Figure 6 c. The fitting of the standard evaluation function yielded an R 2 value of 0.99996 and a slope (k) of −24.103/μm. The theoretical sensitivity of the defocus detection system is 1/k, which is approximately 0.041 μm.
Simulation analysis and Figure 6 c indicate that the focus evaluation function exhibits monotonicity and a high degree of linearity. To evaluate the system’s single iteration focusing performance, a defocus quantification experiment was conducted with increments of 5 μm across a 50 μm range, involving 11 predetermined defocus levels. The system determined the defocus magnitude from a single measurement using the evaluation function. To enhance the validity of the results, the process was replicated three times. As illustrated in Figure 7 a, the discrepancies in identification for various defocus amounts were all within a ±0.1 μm deviation, confined to a ±40 μm range. The system’s single-iteration operating range extends to ±40 μm, achieving an accuracy of 200 nm.

Focus accuracy test experiment. ( a ) Capture single images under different defocus conditions and calculate the amount of defocus, then determine the deviation from the theoretical value. The red line represents deviations of 0.1 μm and −0.1 μm. ( b ) Step out of focus 78 nm multiple times during continuous detection and record the amount of out−of−focus in real time.
Figure 7 b shows the results of continuous tests in which the objective was moved from negative to positive defocus points in 78 nm increments, with the output of the focusing system’s continuous-time sampling. During continuous-time sampling, the focal plane position of the output fluctuated within ±100 nm, which could be caused by environmental vibration or changes in laser intensity. This limited the focusing accuracy for taking a defocus photograph to 200 nm, which is also consistent with the results shown in Figure 7 a. Nevertheless, the mean fluctuation tracked the shifts in defocus, as denoted by the green data in Figure 7 b. The green data, which represents the averaged resampling outcomes, exhibited a closer alignment with the true defocus levels. This evidence suggests that the continuous measurement mode is capable of discerning defocus with 78 nm accuracy over a ±500 nm span.
The test results shown in Figure 7 a indicate that the system maintains good focusing accuracy within a dynamic range of ±40 μm. Beyond ±40 μm, the accuracy decreases significantly. However, experiments have shown that within a defocusing range of ±100 μm, the maximum deviation does not exceed 10 μm, which is confirmed by the evaluation curve with R = 0.99216 in Figure 6 b. Within the defocus range of ±100 μm, the defocus amount can be reduced to within 10 μm after the first iteration. The second iteration can utilize the defocus amount within a range of ±100 nm. There is a defocus amount within ±500 nm after two iterations; the third multisampling identification iteration shown in Figure 7 b can achieve a focusing accuracy of 78 nm. Therefore, three iterations can achieve a focusing accuracy of 78 nm within a range of ±100 μm. In addition, increasing the number of iterations can further increase the dynamic range of the autofocus.
3.3. Sequencing Experiment
The deep-sea sequencer is contained in a compressive cabin, as shown in Figure 8 a, for long-term, in-situ deployment on the deep-sea floor. A series of deep-sea environment simulations were conducted to assess the impact of temperature fluctuations on the defocus levels of an imaging system within a pressure-resistant chamber for a prototype deep-sea gene sequencer. These simulations were conducted within a high and low-temperature environmental test cabinet (Cqhardy), which replicated conditions ranging from surface to deep-sea temperatures (4 °C to 25 °C).

Temperature experiments in a simulated deep-sea environment. ( a ) Deep-sea gene sequencer. ( b ) Focal plane shift due to ambient temperature changes in the pressure-resistant cavity of a deep-sea, in situ gene sequencer.
As illustrated in Figure 8 b, temperature fluctuations resulted in relative positional shifts between the object plane and the lens, with a maximum displacement of approximately 35 µm. Notably, there was a lag in the system’s response to environmental temperature changes that precluded effective compensation through environmental adjustments.
In our laboratory trials, we emulated the deep-sea’s low-temperature conditions and performed sequencing on an Illumina PhiX Control v3—using a prototype sequencer enabled with critical illumination autofocus—at 4 °C. The autofocused image of a chip segment, shown in Figure 9 a, allowed for clear identification of base positions. The data was processed using background subtraction and Gaussian fitting, resulting in the intensity histogram shown in Figure 9 b. Fluorescence information was characterized by higher grayscale values (average intensity of 24.89), while nonfluorescent background areas had lower grayscale values (average intensity of 0). The signal’s deviation above this background, which follows a normal distribution with a standard deviation (STD) of 2.25 for noise, led to an average signal-to-noise ratio of 13.07. This ratio was calculated by dividing the signal intensity by the noise intensity.

Standard nucleotide fragment sequencing experiment. ( a ) Grayscale histogram of local imaging on the chip. ( b ) Extracted intensity histogram. ( c ) Energy values of the four spectral channels with a single cluster. ( d ) Quality score Q30 values for overall data.
Fluorescence intensity data were extracted using a deep learning approach to obtain base sequences and associated quality metrics. Figure 9 c shows the variation in fluorescence intensity at a given base position across four channels throughout the sequencing process. In gene sequencing, Q30 is used to assess the accuracy of sequencing data. Q30 indicates that approximately 1 in 1000 bases sequenced is likely to be incorrect and that sequencing results are 99.9% accurate. The Q30 value represents the proportion of DNA clusters positions identified at a 99.9% confidence level and is a key quality indicator in gene sequencing. Figure 9 d illustrates the real-time Q30 quality scores. Defocusing significantly reduces image quality and thus significantly reduces the Q30 value. The sequencing process culminated in an impressive Q30 value of 97.36%, proving that accurate focus was maintained during sequencing.
4. Discussion
Testing has demonstrated that the deep-sea gene sequencer can sustain a maximum defocus of 35 μm within its operational temperature range. The device employs an autofocus method that relies on critical illumination, enabling it to achieve a focusing accuracy of 0.2 μm with a single capture and iteration over a defocus range of ±40 μm. This fulfills the stringent demands of deep-sea sequencing applications. The accuracy can be further refined by increasing the number of captures while adding iterations, which broadens the dynamic focus range. The detection limits of current systems are collectively determined by the objective lens’s magnification and focal length, the detector’s pixel size, and the width of the field of view.
Moreover, the applicability of this method extends beyond the in-situ use of gene sequencers in deep-sea environments. Researchers have the option to introduce separate wavelength laser illumination designed for focus detection, expanding its compatibility with existing gene sequencers and fluorescence microscopy systems. Additionally, the incorporation of an additional focus detection sensor would enable its integration as a standalone autofocus module for a variety of microscopy systems. Regardless of the shape of the laser illumination, an ideal focus detection signal edge should exhibit a “ridge-shaped” profile. The accuracy of the method is also contingent on the characteristics of the test surface; a smoother surface translates to enhanced accuracy. However, surface reflectivity is marginally relevant since the laser’s output power is significantly greater than that required for detecting focus. In addition, the edge detection algorithm, which is based on the distance between peaks and troughs of grayscale gradients, is an effective way of identifying the edges of defocused images.
Acknowledgments
Yue Wang acknowledges the support from ShuGuang Talents Scheme Award of CIOMP, China.
Supplementary Materials
The following supporting information can be downloaded at: https://www.mdpi.com/article/10.3390/s24155010/s1 , gene sequence information of Illumina PhiX Control v3.
Funding Statement
The National Key R&D Program of China (2021YFC2800300) and the National Natural Science Foundation of China (U21A20395).
Author Contributions
M.G.: Presentation of design proposals, simulations, experiments and writing. Y.W. (Yihui Wu): Proposed the research and its preliminary design, supervised experiments, and writing. Y.W. (Yue Wang): Set up the optical system and writing. F.S.: Researched the experimental methods and set up the thermal cycling device. H.L.: Set up the microfluidic devices and supervised the experiments. W.Z.: Experimental tutoring. Z.S.: LabView programming. S.Z.: Data statistics tutorial. All authors have read and agreed to the published version of the manuscript.
Institutional Review Board Statement
Not applicable.
Informed Consent Statement
Data availability statement, conflicts of interest.
The authors declare no conflicts of interest.
Disclaimer/Publisher’s Note: The statements, opinions and data contained in all publications are solely those of the individual author(s) and contributor(s) and not of MDPI and/or the editor(s). MDPI and/or the editor(s) disclaim responsibility for any injury to people or property resulting from any ideas, methods, instructions or products referred to in the content.

IMAGES
COMMENTS
Everything in the deep ocean is under a great deal of pressure. At any depth in the ocean, the weight of the water above pushes on any object below it. With every foot an object descends into the ocean, more water is pushing down and against it, and more pressure is exerted upon that object. In fact, for every 10 meters traveled deeper into the ...
Deep-sea master-slave hydraulic manipulator is among most commonly tools equipped on remotely-operated vehicles or human occupied vehicles. The design and realization of a 7-Function master-slave hydraulic manipulator can be used in 7000 meters depth is proposed. Linear actuator, rotary actuator and cycloid motor are the three basic modules of the slave arm. A double-screw-pair swing rotary ...
In this paper, a deep-sea large-volume high-pressure chamber is designed. It could simulate up to 6000-m-deep water high-pressure environment. After the theoretical calculation of the overall structure, the finite element software Ansys/Workbench was used to analyze the strength and the sealing performance of the high-pressure chamber.
The spherical pressure hull, as a typical structure in underwater engineering, is a core component that withstands pressure loading in deep-sea high-pressure environment [1]. Scholars worldwide have focus on it for decades, even accumulating a wealth of theoretical, experimental, and manufacturing experience and achievements.
To illustrate the effect of water pressure on air spaces in the deep sea, a styrofoam head was carried by a submarine, from the surface down into the ocean t...
As other underwater equipment especially deep-sea equipment, pressure experiment is a very important and necessary step in the manipulator's development. This paper briefly presents the whole system of 7000 meters depth-rating hydraulic manipulator and the 7000m pressure experiment, and finally, actually application
In the process of ocean exploration and research with an average depth of 3800 m, accurate pressure measurements, such as wave tide measurement, 1,2 tsunami warning system, 3-5 levelness adjustment of oil and gas exploitation platform, 6,7 and precise positioning of underwater robots in the deep sea, 8 are required. Therefore, the pressure sensor should not only measure the larger pressure ...
The design and realization of a 7-Function master-slave deep-sea hydraulic manipulator can be used in 7000 meters depth is proposed. Linear actuator, rotary actuator and cycloid motor are the three basic modules of the slave arm. To achieve smooth control result, PI control algorithm with variable gains is applied on the control of the slave arm.
IV. CONCLUSION The design and 7000m pressure experiment of a deep-sea hydraulic manipulator are proposed in this paper. Some phenomena never occurred in running on land arose in 30MPa and 40MPa ...
The experiment is to validate (1)the slave controller and. salve arm could endure hydraulic pressur e correspond with. 0~7000m ocean pressure, (2)no structure and component. of the slav e ...
The High Pressure Experiments That Made D-Day. What happens to the body in the deep sea? You need oxygen to survive, but too much oxygen can be deadly. Also, if you rise to the surface too quickly ...
In the present pressure experiments, the experimenter rotates a disc on the microscope assembly to measure the BR of a spindle in focus as the cell proceeds through its mitosis, or as pressure is varied. ... Assessing microbial processes in deep-sea hydrothermal systems via incubations at in situ temperature and pressure. Deep-Sea Res I 115:221 ...
Implosion may occur when a hollow pressure structure with geometric imperfections works in deep-sea environments. Therefore, the implosion phenomenon and failure mechanisms of a titanium alloy spherical pressure hull are investigated by experiments and developed numerical methods in ultra-high-pressure water conditions.
In recent decades, the observation of the deep ocean layer between 2000 and 4000 m isobaths 1,2, which would help improve estimates of the ocean heat, freshwater content, and sea level rise, has ...
Timeline of the incubation experiment. Deep-sea samples were recovered at 3,000 m-depth and incubated at in situ pressure conditions (HP) or after decompression at atmospheric ... "Life under pressure. Deep-sea microbial ecology," in Life as we know it. Series: Cellular Origin and Life in Extreme Habitats and Astrobiology, vol. 10. ed J ...
Deep-sea master-slave hydraulic manipulator is among most commonly tools equipped on remotely-operated vehicles or human occupied vehicles. The design and realization of a 7-Function master-slave hydraulic manipulator can be used in 7000 meters depth is proposed. Linear actuator, rotary actuator and cycloid motor are the three basic modules of the slave arm. A double-screw-pair swing rotary ...
One major challenge with creating the experimental setup was maintaining the same pressure found 0.6 miles (1 kilometers) below the ocean surface — about 100 times the air pressure at sea level. Previous experiments have tested similar chemical reactions in individual high-pressure chambers, but White and her colleagues wanted to more fully ...
What happens to flesh and bone when it's subjected to extreme pressure of the deep sea? Does it destroy it, or would it be good for meat tenderizing? Ocean p...
Finally, based on the experimental prototype that has been built and under the circumstance of simulating the deep-sea pressure, we carry out function and performance experiment of the system according to the experimental method and procedure that have been designed in advance. ... The results of pressure experiment indicate that the energy ...
Abstract: Deep-sea electric manipulator usually adopts internally oil-filled method, and the joint motors are soaked in the oil, so that the oil pressure and the water pressure outside will be basically balanced. However, due to the high pressure in deep sea, the oil viscosity increases, and the viscous frictional resistance will cause the viscous power loss of motor to become bigger.
Deep-sea pressure found to preserve food for microbes in the abyss. A new study from the Danish Center for Hadal Research reports on a series of experiments with exposing marine snow to increasing ...
With more than 50 years of experience in offshore and marine technologies, Southwest Research Institute (SwRI) offers a wide variety of services to meet the need for deep ocean pressure simulation testing. These pressure simulation services provide a final check of quality and operational integrity for clients including: Oil producers Manufacturers of subsea components Pipeline manufacturers U.S.
Finally, the 5 L initial samples were concentrated to 50 mL and VC samples were stored at 4 °C until further experiments. 2.2. Deep-sea environment simulation. The virus was diluted with 30 kDa filtered deep sea water ... A pressure of 30 MPa for 2 years was insufficient to totally inactivate the S-CBP1 ...
Rare-earth mud and manganese nodules coexist on the seafloor around Minamitorishima Island. To investigate the feasibility of a pulp-lift system that can ensure economic efficiency by pumping manganese using rare-earth mud as the working fluid, we conducted pulp-lift experiments at a head of 5.0 m using a squeeze pump for mortar pumping. In the study, we used carboxymethylcellulose (CMC) as ...
3.3. Sequencing Experiment. The deep-sea sequencer is contained in a compressive cabin, as shown in Figure 8 a, for long-term, in-situ deployment on the deep-sea floor. A series of deep-sea environment simulations were conducted to assess the impact of temperature fluctuations on the defocus levels of an imaging system within a pressure ...