Thank you for visiting nature.com. You are using a browser version with limited support for CSS. To obtain the best experience, we recommend you use a more up to date browser (or turn off compatibility mode in Internet Explorer). In the meantime, to ensure continued support, we are displaying the site without styles and JavaScript.
- View all journals
- Explore content
- About the journal
- Publish with us
- Sign up for alerts
- Published: 16 February 2023

Sulfate reduction accelerates groundwater arsenic contamination even in aquifers with abundant iron oxides
- Athena A. Nghiem ORCID: orcid.org/0000-0001-9135-0804 1 , 2 nAff7 nAff8 ,
- Henning Prommer ORCID: orcid.org/0000-0002-8669-8184 3 , 4 ,
- M. Rajib H. Mozumder ORCID: orcid.org/0000-0002-2243-1245 1 , 2 , 5 ,
- Adam Siade ORCID: orcid.org/0000-0003-3840-5874 3 , 4 ,
- James Jamieson ORCID: orcid.org/0000-0002-6161-5020 3 , 4 ,
- Kazi Matin Ahmed 6 ,
- Alexander van Geen 2 &
- Benjamin C. Bostick ORCID: orcid.org/0000-0002-7513-6469 2
Nature Water volume 1 , pages 151–165 ( 2023 ) Cite this article
1520 Accesses
12 Citations
7 Altmetric
Metrics details
- Element cycles
- Geochemistry
- Pollution remediation
Groundwater contamination by geogenic arsenic is a global problem affecting nearly 200 million people. In South and Southeast Asia, a cost-effective mitigation strategy is to use oxidized low-arsenic aquifers rather than reduced high-arsenic aquifers. Aquifers with abundant oxidized iron minerals are presumably safeguarded against immediate arsenic contamination, due to strong sorption of arsenic onto iron minerals. However, preferential pumping of low-arsenic aquifers can destabilize the boundaries between these aquifers, pulling high-arsenic water into low-arsenic aquifers. We investigate this scenario in a hybrid field-column experiment in Bangladesh where naturally high-arsenic groundwater is pumped through sediment cores from a low-arsenic aquifer, and detailed aqueous and solid-phase measurements are used to constrain reactive transport modelling. Here we show that elevated groundwater arsenic concentrations are induced by sulfate reduction and the predicted formation of highly mobile, poorly sorbing thioarsenic species. This process suggests that contamination of currently pristine aquifers with arsenic can occur up to over 1.5 times faster than previously thought, leading to a deterioration of urgently needed water resources.
This is a preview of subscription content, access via your institution
Access options
Subscribe to this journal
Receive 12 digital issues and online access to articles
111,21 € per year
only 9,27 € per issue
Buy this article
- Purchase on SpringerLink
- Instant access to full article PDF
Prices may be subject to local taxes which are calculated during checkout
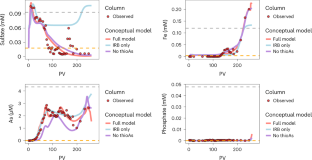
Similar content being viewed by others
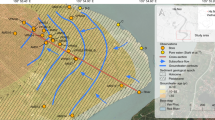
The river–groundwater interface as a hotspot for arsenic release
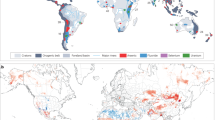
Arsenic and other geogenic contaminants in global groundwater
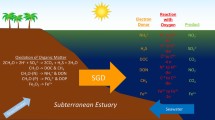
SGD-OD: investigating the potential oxygen demand of submarine groundwater discharge in coastal systems
Data availability.
The column transport parameters and geochemical solute data for the influent groundwater and all column effluent used for modelling have been previously published and can be found in Mozumder et al. 29 . The solid-phase data for the unaltered sediment can also be found in Mozumder et al. 29 . The mineralogy data at the end of the experiment for the Pleistocene columns are in Supplementary Tables 1 and 2 . The normalized XAS spectra and standards used for both Fe EXAFS and As XANES are also available in in the additional Supplementary Information files. The data compilation of groundwater in Bangladesh is a subset of data publicly available at https://doi.org/10.7916/d8-zenj-yx36 .
Code availability
ORTi3D was used for the initial model construction and manual model development and calibration phase ( https://orti3d.ensegid.fr/ ). ORTi3D is an open source software that is a graphical user interface for the public domain codes MODFLOW 78 , MT3DMS 81 and PHT3D 79 . MODFLOW is available from the United States Geological Survey (USGS) at https://www.usgs.gov/software/software-modflow , and PHT3D is available from www.phtd.org . PEST++ for model calibration is also publicly available ( https://github.com/usgs/pestpp ). Finally, the input data files for the reactive transport model are publicly available at https://doi.org/10.7916/945c-9w77 .
Gleeson, T. et al. Groundwater sustainability strategies. Nat. Geosci. 3 , 378–379 (2010).
Article CAS Google Scholar
Ponthieu, M., Juillot, F., Hiemstra, T., van Riemsdijk, W. H. & Benedetti, M. F. Metal ion binding to iron oxides. Geochim. Cosmochim. Acta 70 , 2679–2698 (2006).
McKenzie, R. M. The adsorption of lead and other heavy metals on oxides of manganese and iron. Aust. J. Soil Res. 18 , 61–73 (1980).
Fendorf, S., Michael, H. A. & van Geen, A. Spatial and temporal variations of groundwater arsenic in South and Southeast Asia. Science 328 , 1123–1127 (2010).
Article CAS PubMed Google Scholar
Islam, F. S. et al. Role of metal-reducing bacteria in arsenic release from Bengal delta sediments. Nature 430 , 68–71 (2004).
Smedley, P. L. & Kinniburgh, D. G. A review of the source, behaviour and distribution of arsenic in natural waters. Appl. Geochem. 17 , 517–568 (2002).
Nickson, R. T., Mcarthur, J. M., Ravenscroft, P., Burgess, W. G. & Ahmed, K. M. Mechanism of arsenic release to groundwater, Bangladesh and West Bengal. Appl. Geochem. 15 , 403–413 (2000).
Podgorski, J. & Berg, M. Global threat of arsenic in groundwater. Science 368 , 845–850 (2020).
Postma, D. et al. Mobilization of arsenic and iron from Red River floodplain sediments, Vietnam. Geochim. Cosmochim. Acta 74 , 3367–3381 (2010).
Polizzotto, M. L., Harvey, C. F., Sutton, S. R. & Fendorf, S. Processes conducive to the release and transport of arsenic into aquifers of Bangladesh. Proc. Natl. Acad. Sci. USA 102 , 18819–18823 (2005).
Article CAS PubMed PubMed Central Google Scholar
Burton, E. D., Johnston, S. G. & Planer-Friedrich, B. Coupling of arsenic mobility to sulfur transformations during microbial sulfate reduction in the presence and absence of humic acid. Chem. Geol. 343 , 12–24 (2013).
Planer-Friedrich, B., Schaller, J., Wismeth, F., Mehlhorn, J. & Hug, S. J. Monothioarsenate occurrence in Bangladesh groundwater and its removal by ferrous and zero-valent iron technologies. Environ. Sci. Technol. 52 , 5931–5939 (2018).
Stucker, V. K., Silverman, D. R., Williams, K. H., Sharp, J. O. & Ranville, J. F. Thioarsenic species associated with increased arsenic release during biostimulated subsurface sulfate reduction. Environ. Sci. Technol. 48 , 13367–13375 (2014).
Sun, J., Quicksall, A. N., Chillrud, S. N., Mailloux, B. J. & Bostick, B. C. Arsenic mobilization from sediments in microcosms under sulfate reduction. Chemosphere 153 , 254–261 (2016).
Kocar, B. D., Borch, T. & Fendorf, S. Arsenic repartitioning during biogenic sulfidization and transformation of ferrihydrite. Geochim. Cosmochim. Acta 74 , 980–994 (2010).
Burton, E. D., Johnston, S. G. & Kocar, B. D. Arsenic mobility during flooding of contaminated soil: the effect of microbial sulfate reduction. Environ. Sci. Technol. 48 , 13660–13667 (2014).
van Geen, A. et al. Retardation of arsenic transport through a Pleistocene aquifer. Nature 501 , 204–207 (2013).
Article PubMed PubMed Central Google Scholar
Winkel, L. H. E. et al. Arsenic pollution of groundwater in Vietnam exacerbated by deep aquifer exploitation for more than a century. Proc. Natl. Acad. Sci. USA 108 , 1246–1251 (2011).
Berg, M. et al. Hydrological and sedimentary controls leading to arsenic contamination of groundwater in the Hanoi area, Vietnam: the impact of iron–arsenic ratios, peat, river bank deposits, and excessive groundwater abstraction. Chem. Geol. 249 , 91–112 (2008).
Michael, H. A. & Voss, C. I. Evaluation of the sustainability of deep groundwater as an arsenic-safe resource in the Bengal Basin. Proc. Natl Acad. Sci. USA 105 , 8531–8536 (2008).
Jamil, N. B. et al. Effectiveness of different approaches to arsenic mitigation over 18 years in Araihazar, Bangladesh: implications for national policy. Environ. Sci. Technol. 53 , 5596–5604 (2019).
Hoque, M. A., Hoque, M. M. & Ahmed, K. M. Declining groundwater level and aquifer dewatering in Dhaka metropolitan area, Bangladesh: causes and quantification. Hydrogeol. J. 15 , 1523–1534 (2007).
Article Google Scholar
Wallis, I. et al. The river–groundwater interface as a hotspot for arsenic release. Nat. Geosci. 13 , 288–295 (2020).
Khan, M. R. et al. Megacity pumping and preferential flow threaten groundwater quality. Nat. Commun. 7 , 1–8 (2016).
Prommer, H., Sun, J. & Kocar, B. D. Using reactive transport models to quantify and predict groundwater quality. Elements 15 , 87–92 (2019).
Siade, A. J., Bostick, B. C., Cirpka, O. A. & Prommer, H. Unraveling biogeochemical complexity through better integration of experiments and modeling. Environ. Sci. Process. Impacts 23 , 1825–1833 (2021).
Couture, R. M. et al. Sorption of arsenite, arsenate, and thioarsenates to iron oxides and iron sulfides: a kinetic and spectroscopic investigation. Environ. Sci. Technol. 47 , 5652–5659 (2013).
Suess, E. & Planer-Friedrich, B. Thioarsenate formation upon dissolution of orpiment and arsenopyrite. Chemosphere 89 , 1390–1398 (2012).
Mozumder, M. R. H. et al. Similar retardation of arsenic in gray Holocene and orange Pleistocene sediments: evidence from field-based column experiments in Bangladesh. Water Res. 183 , 116081 (2020).
Sun, J. et al. Simultaneously quantifying ferrihydrite and goethite in natural sediments using the method of standard additions with X-ray absorption spectroscopy. Chem. Geol. 476 , 248–259 (2018).
Nghiem, A. A. et al. Aquifer-scale observations of iron redox transformations in arsenic-impacted environments to predict future contamination. Environ. Sci. Technol. Lett. 7 , 916–922 (2020).
Buschmann, J. & Berg, M. Impact of sulfate reduction on the scale of arsenic contamination in groundwater of the Mekong, Bengal and Red River deltas. Appl. Geochem. 24 , 1278–1286 (2009).
Keimowitz, A. R. et al. Laboratory investigations of enhanced sulfate reduction as a groundwater arsenic remediation strategy. Environ. Sci. Technol. 41 , 6718–6724 (2007).
Kirk, M. F. et al. Bacterial sulfate reduction limits natural arsenic contamination in groundwater. Geology 32 , 953–956 (2004).
Mozumder, M. R. H. et al. Origin of groundwater arsenic in a rural pleistocene aquifer in Bangladesh depressurized by distal municipal pumping. Water Resour. Res. 56 , 1–26 (2020).
Prommer, H., Grassi, M. E., Davis, A. C. & Patterson, B. M. Modeling of microbial dynamics and geochemical changes in a metal bioprecipitation experiment. Environ. Sci. Technol. 41 , 8433–8438 (2007).
Druhan, J. L. et al. Timing the onset of sulfate reduction over multiple subsurface acetate amendments by measurement and modeling of sulfur isotope fractionation. Environ. Sci. Technol. 46 , 8895–8902 (2012).
Jakobsen, R. & Postma, D. Redox zoning, rates of sulfate reduction and interactions with Fe-reduction and methanogenesis in a shallow sandy aquifer, Romo, Denmark. Geochim. Cosmochim. Acta 63 , 137–151 (1999).
Postma, D. & Jakobsen, R. Redox zonation: equilibrium constraints on the Fe (III)/ SO4-reduction interface. Geochim. Cosmochim. Acta 60 , 3169–3175 (1996).
Poulton, S. W., Krom, M. D. & Raiswell, R. A revised scheme for the reactivity of iron (oxyhydr)oxide minerals towards dissolved sulfide. Geochim. Cosmochim. Acta 68 , 3703–3715 (2004).
Thamdrup, B., Finster, K., Hansen, J. W. & Bak, F. Bacterial disproportionation of elemental sulfur coupled to chemical reduction of iron or manganese. Appl. Environ. Microbiol. 59 , 101–108 (1993).
Hansel, C. M. et al. Dominance of sulfur-fueled iron oxide reduction in low-sulfate freshwater sediments. ISME J. 9 , 2400–2412 (2015).
Wind, T. & Conrad, R. Sulfur compounds, potential turnover of sulfate and thiosulfate, and numbers of sulfate-reducing bacteria in planted and unplanted paddy soil. FEMS Microbiol. Ecol. 18 , 257–266 (1995).
Manning, B. A. & Goldberg, S. Modeling competitive adsorption of arsenate with phosphate and molybdate on oxide minerals. Soil Sci. Soc. Am. J. 60 , 121–131 (1996).
Dixit, S. & Hering, J. G. Comparison of arsenic(V) and arsenic(III) sorption onto iron oxide minerals: Implications for arsenic mobility. Environ. Sci. Technol. 37 , 4182–4189 (2003).
Hansel, C. M., Benner, S. G. & Fendorf, S. Competing Fe (II)-induced mineralization pathways of ferrihydrite. Environ. Sci. Technol. 39 , 7147–7153 (2005).
Sun, J., Chillrud, S. N., Mailloux, B. J. & Bostick, B. C. In situ magnetite formation and long-term arsenic immobilization under advective flow conditions. Environ. Sci. Technol. 50 , 10162–10171 (2016).
Coker, V. S. et al. XAS and XMCD evidence for species-dependent partitioning of arsenic during microbial reduction of ferrihydrite to magnetite. Environ. Sci. Technol. 40 , 7745–7750 (2006).
Kumar, N. et al. Redox heterogeneities promote thioarsenate formation and release into groundwater from low arsenic sediments. Environ. Sci. Technol. 54 , 3237–3244 (2020).
Suess, E., Wallschläger, D. & Planer-Friedrich, B. Stabilization of thioarsenates in iron-rich waters. Chemosphere 83 , 1524–1531 (2011).
Wang, J. et al. Thiolated arsenic species observed in rice paddy pore waters. Nat. Geosci. 13 , 282–287 (2020).
Planer-Friedrich, B., London, J., Mccleskey, R. B., Nordstrom, D. K. & Wallschläger, D. Thioarsenates in geothermal waters of yellowstone National Park: determination, preservation, and geochemical importance. Environ. Sci. Technol. 41 , 5245–5251 (2007).
O’Day, P. A., Vlassopoulos, D., Root, R. & Rivera, N. The influence of sulfur and iron on dissolved arsenic concentrations in the shallow subsurface under changing redox conditions. Proc. Natl Acad. Sci. USA 101 , 13703–13708 (2004).
Planer-Friedrich, B. et al. Anaerobic chemolithotrophic growth of the haloalkaliphilic bacterium strain MLMS-1 by disproportionation of monothioarsenate. Environ. Sci. Technol. 49 , 6554–6563 (2015).
Stauder, S., Raue, B. & Sacher, F. Thioarsenates in sulfidic waters. Environ. Sci. Technol. 39 , 5933–5939 (2005).
Helz, G. R. & Tossell, J. A. Thermodynamic model for arsenic speciation in sulfidic waters: a novel use of ab initio computations. Geochim. Cosmochim. Acta 72 , 4457–4468 (2008).
Vlassopoulos, D., Bessinger, B. & O’day, P. A. Aqueous solubility of As2S3 and thermodynamic stability of thioarsenites. Water-Rock Interact . 823–826 (CRC Press, 2010).
Wilkin, R. T. et al. Thioarsenite detection and implications for arsenic transport in groundwater. Environ. Sci. Technol. 53 , 11684–11693 (2019).
Planer-Friedrich, B., Suess, E., Scheinost, A. C. & Wallschläger, D. Arsenic speciation in sulfidic waters: reconciling contradictory spectroscopic and chromatographic evidence. Anal. Chem. 82 , 10228–10235 (2010).
Planer-Friedrich, B. Comment on ‘Thioarsenite detection and implications for arsenic transport in groundwater’. Environ. Sci. Technol. 54 , 7730–7731 (2020).
Knappett, P. S. K. et al. Vulnerability of low-arsenic aquifers to municipal pumping in Bangladesh. J. Hydrol. 539 , 674–686 (2016).
Kazmierczak, J. et al. Groundwater arsenic content in quaternary aquifers of the Red River delta, Vietnam, controlled by the hydrogeological processes. J. Hydrol. 609 , 127778 (2022).
Michael, H. A. & Voss, C. I. Controls on groundwater flow in the Bengal Basin of India and Bangladesh: regional modeling analysis. Hydrogeol. J. 17 , 1561–1577 (2009).
Shamsudduha, M., Taylor, R. G. & Chandler, R. E. A generalized regression model of arsenic variations in the shallow groundwater of Bangladesh. Water Resour. Res. 51 , 685–703 (2015).
Planer-Friedrich, B. et al. Organic carbon mobilization in a Bangladesh aquifer explained by seasonal monsoon-driven storativity changes. Appl. Geochem. 27 , 2324–2334 (2012).
Suess, E., Mehlhorn, J. & Planer-Friedrich, B. Anoxic, ethanolic, and cool—an improved method for thioarsenate preservation in iron-rich waters. Appl. Geochem. 62 , 224–233 (2015).
Zheng, Y. et al. Redox control of arsenic mobilization in Bangladesh groundwater. Appl. Geochem. 19 , 201–214 (2004).
Burton, E. D., Johnston, S. G. & Bush, R. T. Microbial sulfidogenesis in ferrihydrite-rich environments: effects on iron mineralogy and arsenic mobility. Geochim. Cosmochim. Acta 75 , 3072–3087 (2011).
Saalfield, S. L. & Bostick, B. C. Changes in iron, sulfur, and arsenic speciation associated with bacterial sulfate reduction in ferrihydrite-rich systems. Environ. Sci. Technol. 43 , 8787–8793 (2009).
Harvey, C. F. et al. Arsenic mobility and groundwater extraction in Bangladesh. Science 298 , 1602–1606 (2002).
Besold, J. et al. Monothioarsenate transformation kinetics determining arsenic sequestration by sulfhydryl groups of peat. Environ. Sci. Technol. 52 , 7317–7326 (2018).
Eberle, A. et al. Potential of high pH and reduced sulfur for arsenic mobilization—insights from a Finnish peatland treating mining waste water. Sci. Total Environ. 758 , 143689 (2021).
Van Geen, A. et al. Spatial variability of arsenic in 6000 tube wells in a 25 km 2 area of Bangladesh. Water Resour. Res. 39 , 1–16 (2003).
Google Scholar
Ahsan, H. et al. Health Effects of Arsenic Longitudinal Study (HEALS): description of a multidisciplinary epidemiologic investigation. J. Expo. Sci. Environ. Epidemiol. 16 , 191–205 (2006).
Newville, M. Larch: an analysis package for XAFS and related spectroscopies. J. Phys. Conf. Ser. 430 , 012007 (2013).
Webb, S. M. SIXPack a graphical user interface for XAS analysis using IFEFFIT. Phys. Scr . https://doi.org/10.1238/Physica.Topical.115a01011 (2005).
Shoenfelt, E. M., Winckler, G., Lamy, F., Anderson, R. F. & Bostick, B. C. Highly bioavailable dust-borne iron delivered to the Southern Ocean during glacial periods. Proc. Natl Acad. Sci. USA 115 , 201809755 (2018).
Harbaugh, A. W. MODFLOW-2005: the U. S. Geological survey modular ground-water mode—the ground-water flow process. In6. modeling techniques, chapter A16. US Geol. Surv. Rest. 92 , 1–134 (2005).
Prommer, H., Barry, D. A. & Zheng, C. MODFLOW/MT3DMS-based reactive multicomponent transport modeling. Ground Water 41 , 247–257 (2003).
Parkhurst, D. L. & Appelo, C. A. J. User’s Guide To PHREEQC (version 2)—a computer program for speciation, and inverse geochemical calculations. U.S. Geol. Surv. Water-Resources Investig. Rep. 326 , 99–4259 (1999).
Zheng, C. & Wang, P. MT3DMS: A modular three-dimensional multispeces transport model for simulation of advection, dispersion, and chemical reactions of contaminants in groundwater systems. Documentation and User’s Guide. U.S. Army Corps Eng . https://doi.org/10.1007/978-1-4419-6530-1 (1998).
White, J. T., Hunt, R. J., Fienen, M. N. & Doherty, J. E. Approaches to highly parameterized inversion: PEST++ Version 5, a software suite for parameter estimation, uncertainty analysis, management optimization and sensitivity analysis. U.S. Geol. Surv. Tech. Methods 7C26 https://doi.org/10.3133/tm7C26 (2020).
Siade, A. J., Rathi, B., Prommer, H., Welter, D. & Doherty, J. Using heuristic multi-objective optimization for quantifying predictive uncertainty associated with groundwater flow and reactive transport models. J. Hydrol. 577 , 123999 (2019).
Sun, J. et al. Model-based analysis of arsenic immobilization via iron mineral transformation under advective flows. Environ. Sci. Technol. 52 , 9243–9253 (2018).
Rawson, J. et al. Quantifying reactive transport processes governing arsenic mobility after injection of reactive organic carbon into a bengal delta aquifer. Environ. Sci. Technol. 51 , 8471–8480 (2017).
Dzombak, D. A. & Morel, F. M. M. Surface Complexation Modeling: Hydrous Ferric Oxide (John Wiley & Sons, 1990).
Stollenwerk, K. G. et al. Arsenic attenuation by oxidized aquifer sediments in Bangladesh. Sci. Total Environ. 379 , 133–150 (2007).
Hinkle, M. A. G., Wang, Z., Giammar, D. E. & Catalano, J. G. Interaction of Fe(II) with phosphate and sulfate on iron oxide surfaces. Geochim. Cosmochim. Acta 158 , 130–146 (2015).
Swedlund, P. J. & Webster, J. G. Cu and Zn ternary surface complex formation with so4 on ferrihydrite and schwertmannite. Appl. Geochem. 16 , 503–511 (2001).
Boland, D. D., Collins, R. N., Miller, C. J., Glover, C. J. & Waite, T. D. Effect of solution and solid-phase conditions on the Fe(II)-accelerated transformation of ferrihydrite to lepidocrocite and goethite. Environ. Sci. Technol. 48 , 5477–5485 (2014).
Barry, D. A. et al. Modelling the fate of oxidisable organic contaminants in groundwater. Adv. Water Resour. 25 , 945–983 (2002).
Zysset, A., Stauffer, F. & Dracos, T. Modeling of reactive groundwater transport governed by biodegradation. Water Resour. Res. 30 , 2423–2434 (1994).
Bethke, C. M., Sanford, R. A., Kirk, M. F., Jin, Q. & Flynn, T. M. The thermodynamic ladder in geomicrobiology. Am. J. Sci. 311 , 183–210 (2011).
Jin, Q. & Bethke, C. M. A new rate law describing microbial respiration. Appl. Environ. Microbiol. 69 , 2340–2348 (2003).
Morel, F. M. M. & Hering, J. G. Principles and Applications of Aquatic Chemistry (John Wiley & Sons, 1993).
Lasaga, A. C. Kinetic Theory in the Earth Sciences (Princeton Univ. Press, 1998).
Prommer, H., Anneser, B., Rolle, M., Einsiedl, F. & Griebler, C. Biogeochemical and isotopic gradients in a BTEX/PAH contaminant plume: model-based interpretation of a high-resolution field data set. Environ. Sci. Technol. 43 , 8206–8212 (2009).
Pedersen, H. D., Postma, D., Jakobsen, R. & Larsen, O. Fast transformation of iron oxyhydroxides by the catalytic action of aqueous Fe(II). Geochim. Cosmochim. Acta 69 , 3967–3977 (2005).
Cornell, R. M. & Schwertmann, U. The iron oxides: structure, properties, reactions, occurrences and uses. Techniques 39 , 9–12 (2003).
Holmkvist, L., Ferdelman, T. G. & Jørgensen, B. B. A cryptic sulfur cycle driven by iron in the methane zone of marine sediment (Aarhus Bay, Denmark). Geochim. Cosmochim. Acta 75 , 3581–3599 (2011).
Canfield, D. E. et al. A cryptic sulfur cycle in oxygen-minimum-zone waters off the Chilean coast. Science 330 , 1375–1378 (2010).
Ng, G. H. C. et al. Microbial and reactive transport modeling evidence for hyporheic flux-driven cryptic sulfur cycling and anaerobic methane oxidation in a sulfate-impacted wetland-stream system. J. Geophys. Res. Biogeosci. 125 , 1–25 (2020).
Zheng, Y. et al. Geochemical and hydrogeological contrasts between shallow and deeper aquifers in two villages of Araihazar, Bangladesh: implications for deeper aquifers as drinking water sources. Geochim. Cosmochim. Acta 69 , 5203–5218 (2005).
Download references
Acknowledgements
The experimental study was supported by the US National Institute of Environmental Health Sciences (NIEHS) grants ES0101349, ES009089 (B.C.B and A.v.G.) and P42ES033719 (B.C.B. and H.P.), US National Science Foundation (NSF) grant 1521356 (B.C.B. and A.v.G.) and US Department of Energy (DOE) grant EE0009596 (B.C.B. and H.P.). The computational modelling work was supported by an NSF Graduate Research Opportunities Worldwide (GROW) grant awarded to A.A.N. to conduct research in Western Australia. A.A.N. was also funded by an NSF Graduate Research Fellowship (NSF grant 1644869). Use of the Stanford Synchrotron Radiation Lightsource, SLAC National Accelerator Laboratory, is supported by the US Department of Energy, Office of Science, Office of Basic Energy Sciences under contract no. DE-AC02-76SF00515. Computing resources, including high-performance computing, were provided by CSIRO Australia. The data for part of this work were compiled as a part of the Characterizing Global Variability in Groundwater Arsenic Working Group supported by the John Wesley Powell Center for Analysis and Synthesis, funded by the US Geological Survey. We also thank M. Selim, M. Atikul Islam, E. Shoenfelt Troein, T. Ellis, B. Mailloux and I. Choudhury for their contributions to the original experimental work. We also thank M. Stahl and D. Postma for their useful discussions.
Author information
Athena A. Nghiem
Present address: Institute of Biogeochemistry and Pollutant Dynamics, Department of Environmental Systems Science, ETH Zurich, Zurich, Switzerland
Present address: Eawag, Swiss Federal Institute of Aquatic Science and Technology, Dübendorf, Switzerland
Authors and Affiliations
Department of Earth and Environmental Sciences, Columbia University, New York, NY, USA
Athena A. Nghiem & M. Rajib H. Mozumder
Lamont-Doherty Earth Observatory, Columbia University, Palisades, NY, USA
Athena A. Nghiem, M. Rajib H. Mozumder, Alexander van Geen & Benjamin C. Bostick
CSIRO Environment, Wembley, Western Australia, Australia
Henning Prommer, Adam Siade & James Jamieson
School of Earth Sciences, University of Western Australia, Perth, Western Australia, Australia
Ramboll Environment & Health, Westford, MA, USA
M. Rajib H. Mozumder
Department of Geology, University of Dhaka, Dhaka, Bangladesh
Kazi Matin Ahmed
You can also search for this author in PubMed Google Scholar
Contributions
A.A.N., H.P. and B.C.B. conceived the reactive transport modelling work based off the column experiment work conceived by M.R.H.M., A.v.G. and B.C.B. The field work and column experiment work were performed by M.R.H.M., B.C.B. and K.M.A.; M.R.H.M. measured and provided data from the column experiment work. A.A.N. and H.P. performed the flow and reactive transport modelling. A.A.N., H.P., J.J. and B.C.B. contributed to the conceptual models underlying the numerical models. A.A.N. performed data curation, data visualization, analysis and model calibration of the reactive transport modelling results. H.P., A.S. and J.J. also contributed to the methodology, software/computing resources, model calibration and validation of the reactive transport modelling. A.S. also performed model sensitivity analysis. A.A.N. provided funding acquisition for the reactive transport modelling work; B.C.B. and A.v.G. provided funding acquisition for the column experiment and field work. A.A.N., H.P. and B.C.B. wrote and prepared the original manuscript, and all authors contributed to the review and editing of the manuscript.
Corresponding authors
Correspondence to Athena A. Nghiem or Benjamin C. Bostick .
Ethics declarations
Competing interests.
The authors declare no competing interests.
Peer review
Peer review information.
Nature Water thanks Rasmus Jakobsen, Britta Planer-Friedrich and the other, anonymous, reviewer(s) for their contribution to the peer review of this work.
Additional information
Publisher’s note Springer Nature remains neutral with regard to jurisdictional claims in published maps and institutional affiliations.
Supplementary information
Supplementary information.
Supplementary text, Figs. 1–15, Tables 1–9 and references.
Reporting Summary
Supplementary data 1.
Directory containing normalized Fe EXAFS and As XANES spectra.
Supplementary Data 2
Excel file containing root mean squared error for all models.
Rights and permissions
Springer Nature or its licensor (e.g. a society or other partner) holds exclusive rights to this article under a publishing agreement with the author(s) or other rightsholder(s); author self-archiving of the accepted manuscript version of this article is solely governed by the terms of such publishing agreement and applicable law.
Reprints and permissions
About this article
Cite this article.
Nghiem, A.A., Prommer, H., Mozumder, M.R.H. et al. Sulfate reduction accelerates groundwater arsenic contamination even in aquifers with abundant iron oxides. Nat Water 1 , 151–165 (2023). https://doi.org/10.1038/s44221-022-00022-z
Download citation
Received : 19 August 2022
Accepted : 19 December 2022
Published : 16 February 2023
Issue Date : February 2023
DOI : https://doi.org/10.1038/s44221-022-00022-z
Share this article
Anyone you share the following link with will be able to read this content:
Sorry, a shareable link is not currently available for this article.
Provided by the Springer Nature SharedIt content-sharing initiative
This article is cited by
- Abhijit Mukherjee
- Poulomee Coomar
- Avner Vengosh
Nature Reviews Earth & Environment (2024)
Sulfur being an overlooked promoter of groundwater arsenic contamination
- Britta Planer-Friedrich
Nature Water (2023)
Quick links
- Explore articles by subject
- Guide to authors
- Editorial policies
Sign up for the Nature Briefing newsletter — what matters in science, free to your inbox daily.


An official website of the United States government
The .gov means it’s official. Federal government websites often end in .gov or .mil. Before sharing sensitive information, make sure you’re on a federal government site.
The site is secure. The https:// ensures that you are connecting to the official website and that any information you provide is encrypted and transmitted securely.
- Publications
- Account settings
The PMC website is updating on October 15, 2024. Learn More or Try it out now .
- Advanced Search
- Journal List
- HHS Author Manuscripts

COLUMN VERSUS BATCH METHODS FOR MEASURING PFOS AND PFOA SORPTION TO GEOMEDIA
Sarah van glubt.
1 Environmental Science Department, University of Arizona, Tucson, AZ 85721, United States;
Mark L. Brusseau
2 Key Lab of Marine Environmental Science and Ecology, Ministry of Education, College of Environmental Science and Engineering, Ocean University of China, Qingdao, P.R. China;
Dandan Huang
3 School of Water Resources & Environment, China University of Geosciences, Beijing, P.R. China;
4 Department of Plant & Environmental Sciences, New Mexico State University, Las Cruces, New Mexico, United States
Kenneth C. Carroll
Credit Author Statement
Mark L. Brusseau: Conceptualization, Methodology, Resources, Data Analysis, Writing- Original draft preparation, Supervision.
Ni Yan: Investigation, Writing- Review & Editing.
Dandan Huang: Investigation, Writing- Review & Editing.
Naima Khan: Investigation, Writing- Review & Editing.
Kenneth C. Carroll: Resources, Writing- Review & Editing.
Associated Data
The objective of this study is to compare the consistency between column and batch experiment methods for measuring solid-phase sorption coefficients and isotherms for per and polyfluoroalkyl substances (PFAS). Perfluorooctane sulfonic acid (PFOS) and perfluorooctanoic acid (PFOA) are used as representative PFAS, and experiments are conducted with three natural porous media with differing geochemical properties. Column-derived sorption isotherms are generated by conducting multiple experiments with different input concentrations (multi-C 0 method) or employing elution-front integration wherein the entire isotherm is determined from a single breakthrough curve (BTC) elution front. The isotherms generated with the multi-C 0 column method compared remarkably well to the batch isotherms over an aqueous concentration range of 3–4 orders of magnitude. Specifically, the 95% confidence intervals for the individual isotherm variables overlapped, producing statistically identical regressions. The elution-front integration isotherms generally agreed with the batch isotherms, but exhibited noise and systematic deviation at lower concentrations in some cases. All data sets were well described by the Freundlich isotherm model. Freundlich N values ranged from 0.75 to 0.81 for PFOS and was 0.87 for PFOA and are consistent with values reported in the literature for different geomedia. The results of this study indicate that column and batch experiments can measure consistent sorption isotherms and sorption coefficients for PFOS and PFOA when robust experimental setup and data analysis are implemented.
Graphical Abstract

Capsule Statement:
Column and batch methods produced consistent isotherms for PFOS and PFOA sorption by three geomedia of differing geochemical properties.
1. Introduction
Per and polyfluoroalkyl substances (PFAS) have become major emerging contaminants of concern due to their widespread occurrence in the environment and potential for human-health impacts. As a result, there is great interest in their transport and fate behavior in the environment. One process of primary importance for PFAS transport is sorption by soil, sediment, and aquifer material (geological media or geomedia). Research investigating sorption mechanisms, the role of PFAS molecular structure and geomedia properties, and the impact of other factors such as solution chemistry has been ongoing for the past 15 years. The quantification of PFAS sorption through the determination of equilibrium sorption coefficients has also been a focus of attention.
There are two standard means by which to quantify sorption, batch experiments and miscible-displacement column experiments. Over the past 15 years, close to 100 studies have investigated the sorption of PFAS by geomedia and specific constituents of geomedia. Of these, the vast majority have used batch methods to quantify sorption, with only a very few employing column methods ( Vierke et al., 2014 ; McKenzie et al., 2015 ; Aly et al., 2018 , 2019 ; Lyu et al., 2018 ; Lv et al., 2018 ; Brusseau et al., 2019a , 2019b ; Lyu et al., 2019; Guelfo et al., 2020 ; Lyu and Brusseau, 2020 ; Yan et al., 2020 ). Only two of these studies conducted both column and batch experiments and directly compared the measured K d values. Aly et al. (2018) quantified sorption of PFOS and PFOA for a quartz sand. The K d values obtained from the batch experiment were approximately 10-times smaller than the values determined from the column experiments. Conversely, K d values obtained from column and batch methods matched well for sorption of PFOS by two soils in the study reported by Brusseau et al. (2019a) . Two other studies used batch-derived sorption data to evaluate column experiment results, but did not directly compare K d values between the two methods. Brusseau et al. (2019b) used K oc values determined from batch experiments reported in the literature to predict retardation factors for PFOS and PFOA and compared them to values measured from column experiments. The batch-derived predicted retardation factors were consistent with the measured values obtained from the column experiments for both PFOS and PFOA. Guelfo et al. (2020) used batch-derived equilibrium sorption parameters as input into a model to predict column-experiment transport data for 10 PFAS in 4 porous media. They observed good predictions in some cases and poorer predictions in others, concluding that the batch-derived equilibrium parameters did not provide good representation of transport in some instances. Aly et al. (2019) reported K d data measured with both batch and column experiments for the sorption of 6 PFAS by a soil, but did not compare the two sets of data.
The reason(s) for the differences in results between the PFAS studies is not clear. The question of consistency between column and batch methods has long been a focus of investigation for other types of contaminants (e.g., Schweich et al., 1983 ; Nkedi-Kizza et al., 1987 ; Brusseau and Rao, 1989 ; Bürgisser et al., 1993 ; Grolimund et al., 1995 ; Maraqa et al., 1998 ; Altfelder et al., 2001 ; Wang et al., 2009 ; Jolin et al., 2016 ). Consistency between the two methods has been observed in a number of investigations spanning a wide range of solutes, porous media, and data-analysis methods (e.g., Schneider and Smith, 1968 ; Schweich et al., 1983 ; Nkedi-Kizza et al., 1987 ; Lee et al., 1988 ; MacIntyre et al., 1991 ; Bürgisser et al., 1993 ; Allen et al., 1995 ; Grolimund et al., 1995 ; Carroll et al., 2006 ; Mon et al., 2006 ; Kempf and Brusseau, 2009 ; Wang et al., 2009 ; Jolin et al., 2016 ; Brusseau et al., 2019a ). However, other investigations have reported disparities between the two methods (e.g., Celorie et al., 1989 ; Fesch et al., 1998 ; Maraqa et al., 1998 ; Aly et al., 2018 ). In the majority of cases, the discrepancy can typically be traced to artifacts associated with experiment implementation or data interpretation for one or both methods (e.g., Schweich et al., 1983 ; Brusseau and Rao, 1989 ; Grolimund et al., 1995 ; Altfelder et al., 2001 ; Limousin et al., 2007 ; Wang et al., 2009 ).
As noted above, the vast majority of PFAS sorption studies have been conducted using batch methods. The sorption coefficients determined from these studies may be used for parameterizing models for simulating PFAS transport in soil and groundwater. However, the representativeness of the measured sorption coefficients for the dynamic conditions of water flow and solute transport has not been fully investigated for PFAS. The column experiments in all of the prior comparison studies cited above employed only one influent concentration. Thus, the comparisons between column and batch measurements were limited, and it is not clear if the comparisons would differ for column experiments conducted with different input concentrations. Given the current concern over the presence of PFAS in the environment and the potential for PFAS to undergo more complex sorption behavior, it seems prudent to investigate this issue specifically for PFAS.
The objective of this study is to conduct a comparison of column and batch methods specifically for the sorption of PFOS and PFOA, two PFAS of critical concern. Miscible-displacement column experiments and batch-isotherm experiments are conducted with three natural porous media. The present study employs a robust evaluation by measuring full isotherms from column experiments (multiple input concentrations) and comparing to corresponding batch-derived data measured over a broad range of concentrations. Different methods for determining column-derived K d values and sorption isotherms are employed, and their associated advantages and disadvantages are discussed.
2. Materials and Methods
2.1. materials.
The experiments were conducted with PFOS (CAS# 1763-23-1, Sigma-Aldrich, 98% purity) and PFOA (CAS# 335-67-1, AIKE Reagent, 98% purity) as the representative PFAS. Pentafluorobenzoic acid (99%, Strem Chemicals), which is not a PFAS, was used as the nonreactive tracer (NRT). Two soils and a commercial natural quartz sand (Accusand, Unimin Corp., Le Sueur, MN) were used in the experiments. The media have similar median grain diameters, but different geochemical properties ( Table S1 in the Supplemental Information file ). A synthetic groundwater solution was used for the experiments conducted with the soils. A 0.01 M NaCl solution was used for the experiments conducted with the sand. Details of the soils and synthetic groundwater composition are provided in the Supplemental Information (SI-I) .
2.2. Experiments
Measured sorption data reported in our prior publications were used as one source of data. These include column data for PFOA sorption by the sand ( Lyu et al., 2018 ), column data for PFOS sorption by Vinton and Eustis ( Brusseau et al., 2019a ), and batch isotherm data for PFOS sorption by Vinton and Eustis ( Brusseau et al., 2019a ). New experiments were conducted to provide additional sets of column and batch data for a range of initial/input concentrations. The matrix of experiments is presented in Table S2 .
A brief overview of the methods are presented herein; additional details are provided in the SI . Batch experiments were conducted to measure PFOS and PFOA sorption by the sand. Initial concentrations of 0.01, 0.1, 1, and 10 mg/L were used to match the range employed for a prior study that measured PFOS sorption by Eustis and Vinton soils ( Brusseau et al., 2019a ). These concentrations were selected to be representative of the elevated levels typically observed for soils and groundwater at PFAS sources sites such as fire-training areas and manufacturing facilities ( Schultz et al., 2004 ; Brusseau et al. 2020 ). Miscible-displacement experiments were conducted with the nonreactive tracer to characterize the hydrodynamic properties of the packed columns. Experiments were then conducted with PFOS or PFOA to characterize their retardation and transport. Triplicate experiments were conducted for PFOS transport in the sand for C 0 = 1 and 10 mg/L and for Vinton for C 0 = 10 mg/L (see Table S2 ).
The pentafluorobenzoic acid samples were analyzed by ultraviolet-visible (UV-Vis) spectrophotometry (Shimadzu, model 1601). PFOS and PFOA samples for the batch experiments were analyzed by High Performance Liquid Chromatography tandem mass spectrometry (LCMS). The column-experiment samples were analyzed by LCMS and by the methylene blue active substances (MBAS) assay. An overview of the analytical methods is provided in the SI ( section SI – II ), and additional details are provided in Brusseau et al. (2019a) .
2.3. Data Analysis
Concentrations of aqueous solutions in the batch experiments were measured before and after the experiments were completed. A mass balance was used to determine the sorbed concentrations. The measured data were fitted with the Freundlich isotherm model:
where S is the equilibrium sorbed phase concentration (M M −1 ), C is the equilibrium aqueous phase concentration (M L −3 ), K f is the Freundlich sorption coefficient (L 3N M −N ), and N is the Freundlich exponent.
Solute concentrations measured for column-effluent samples were used to develop breakthrough curves (BTC) as a function of pore volumes eluted, with pore volumes representing the volume of solution displaced through the column divided by the volume of water-filled pore space within the column. Multiple methods were used to determine retardation factors and subsequently K d and/or S values from the BTC data. One method is based on moment analysis of the full BTC. The first normalized temporal moment (M 1 ) determines the retention time of a solute by:
where M 0 = ∫ 0 ∞ C * dT is the zeroth moment, C* is the relative concentration (with C* = C/C 0 ), C 0 is the input solution concentration (M L −3 ), T is the pore volume or nondimensional time (with T = νt/L), ν is the mean pore-water velocity (L T −1 ; with ν = q/η), q is the Darcy flux (L T −1 ), η is the porosity (−), t is time, and L is the column length. The retardation factor (R) is determined by:
where T 0 is the input pulse in units of pore volume.
The second method is based on determining the T associated with the center of mass of the BTC:
A third method employs frontal analysis to calculate the normalized retention volume, alternatively referred to as the area above the breakthrough curve:
Alternatively, the normalized retention volume can be calculated from the area under the elution front:
where T elution is the pore volume or nondimensional time of the elution front, and T elution =0 occurs at the end of the input pulse.
Multiple methods can be used to determine sorption isotherms from column experiments. The first method, termed the multi-C 0 method, involves conducting a series of experiments with different input concentrations and calculating corresponding K d and S values for each input concentration. The K d is calculated from the measured R by rearranging:
where K d is the solid-phase sorption coefficient (L M −1 ) and ρ b is the bulk density (M L −3 ). A sorbed-phase concentration is then calculated with the known values for K d and C (C 0 ). The R values determined from frontal analysis ( equation 5 ) were used for this method, as some measured data sets comprised only arrival waves. However, as will be discussed in the Results section, R values determined by moment analysis ( equation 4 ) and frontal analysis were consistent for the full-BTC data sets.
An alternative approach to obtain isotherms from column experiments employs integration of the elution front ( DeVault, 1943 ). Sorbed-phase concentrations are determined by:
This provides determination of individual S values corresponding to each effluent C measured for the elution front, producing an isotherm from one column experiment. This method was applied to the experiment with the highest-resolution elution front for each medium. This was C 0 = 7 mg/L for Eustis, C 0 = 10 mg/L for Vinton, and C 0 = 25 mg/L for the sand. For comparison to the other column-derived data, the Freundlich model is fit to the isotherm data and the model parameters are used to determine K d values for column-experiment C 0 values with:
The K d value is used to calculate R ( equation 7 ).
3. Results and Discussion
3.1. btcs and retardation factors.
BTCs for the NRT are observed to be ideal (see Figure S1 in the SI ), as was observed by Brusseau et al. (2019a) . The dispersivities determined from analysis of the NRT data are 0.2, 0.2, and 0.5 cm for the sand, Vinton, and Eustis, respectively. These small values are consistent with the nature of the three media and are indicative of uniformly packed columns minimally influenced by preferential flow.
In contrast to the NRT, the BTCs for PFOS and PFOA are observed to be nonideal, exhibiting asymmetry and tailing. This is illustrated in Figure S1 for PFOS transport in the sand (the BTCs for PFOS transport in the two soils are presented in Brusseau et al., 2019a ). This behavior was also observed by Brusseau et al. (2019a) and was determined to be caused primarily by rate-limited sorption-desorption, with a minor contribution from nonlinear sorption. Mass recovery values for the column experiments range from 92% to 110%. Assuming that PFOS and PFOA are recalcitrant, the recovery variability is presumed to be due to analytical uncertainty.
The retardation factors determined using the different calculation methods were consistent for each set of experiments. For example, the retardation factors determined from the column experiments specifically used to conduct the elution-integration analysis are presented in Table 1 . The R values determined from moment analysis, center of mass, area above the arrival front, and area below the elution front are quite consistent. This consistency is notable because each method uses different portions of the BTC--- moment analysis and center of mass use the entire BTC, whereas area above the arrival front uses only the arrival front, and area below the elution front uses only the elution front. Each portion of the BTC can have different sources and magnitudes of noise or uncertainty. Additionally, the significance of noise associated with C* and T data differs for each method. For example, the integration terms for center of mass, area above the arrival front, and area below the elution front include C* only ( equations 4 , 5 , and 6 ) while the integration term for moment analysis includes both C* and T ( equation 2 ). The excellent consistency among the Rs obtained with the different methods indicates that the BTCs are self-consistent, robust, and of sufficient resolution to characterize sorption. This in turn indicates that the experimental and analysis methods are robust. This robustness check is routinely used for our column experiments.
Retardation factors determined by various methods for PFOS experiments used to measure elution-integration isotherms
Experiment | Recovery (%) | R Moment | R AAAF | R ABEF | R COM | R EFI |
---|---|---|---|---|---|---|
Eustis C = 7 mg/L | 102 | 4.3 | 4.4 | 4.5 | 4.4 | 4.0 |
Vinton C = 10 mg/L | 98.3 | 3.3 | 3.3 | 3.4 | 3.1 | 2.8 |
Sand C = 25 mg/L | 98.3 | 1.6 | 1.6 | 1.7 | 1.6 | 1.4 |
3.2. Sorption Isotherms: Batch and Multi-C 0 Column Experiments
Isotherms determined from batch and multi-C 0 column experiments for PFOS sorption by Eustis soil are shown in Figure 1A . The two sets of data are essentially coincident over a concentration range spanning four orders of magnitude. Additionally, the small 95% confidence intervals for the batch data indicate good replication. Data from both types of experiments are well described by the Freundlich isotherm model, and indicate PFOS undergoes nonlinear sorption with Eustis soil. Notably, the Freundlich regressions from batch, column, and combined batch and column data show good consistency with very little deviation from one another. The 95% confidence intervals for the individual isotherm variables overlap, producing statistically identical regressions.

Isotherms for PFOS and PFOA sorption determined from batch and column experiments. A: PFOS-Eustis soil; B: PFOS-Vinton soil; C: PFOS-Sand; D: PFOA-Sand. Freundlich isotherms are shown for batch, column, and combined batch and column data. 95% confidence intervals are included for all batch data points and select column data points for which triplicate experiments were conducted (see Table S2 ); note that if the error bars are not observed this means that they are smaller than the symbols.
Isotherms determined from batch and column experiments for PFOS sorption by Vinton soil are shown in Figure 1B . The column-derived data are consistent with the batch data. Small 95% confidence intervals for both batch and column data indicate good reproducibility. As observed for Eustis soil, PFOS sorption by Vinton soil is well described by the Freundlich model over a concentration range spanning more than three orders of magnitude.
Isotherms for PFOS and PFOA sorption by the sand are presented in Figures 1C and and1D, 1D , respectively. The batch and column data exhibit similar consistency and robustness as observed for the prior systems. The individual Freundlich isotherms are essentially coincident for PFOS. Conversely, some disparity between the batch and column regressions is observed for PFOA sorption by sand at the lower concentration range. However, the 95% confidence intervals for the batch isotherm data span the column regression. It is reasonable that the data set with the lowest magnitude of sorption is the one that exhibits the greatest degree of uncertainty.
Previously published sorption data for trichloroethene (TCE), a representative hydrophobic organic contaminant (HOC), are presented to provide a comparison of the methods for a contaminant with differing chemical properties. TCE sorption isotherms with Eustis soil determined from batch and column experiments are shown in Figure S2 . As observed for the PFOS-Eustis isotherms, sorption isotherms for TCE from batch and column experiments are essentially coincident and well described by the Freundlich model across a concentration range of several orders of magnitude.
The column and batch measurements provide remarkably consistent sorption isotherms with small variability for all solutes and media studied herein. The systems tested represent three geomedia with varying geochemical properties, two representative PFAS, and one HOC. These results conclusively demonstrate that equilibrium sorption coefficients (K d , K f , N) measured with the miscible-displacement column experiments are consistent with batch-derived measurements. It is notable that this consistency is observed for PFAS transport data (BTCs) that are influenced by both rate-limited and nonlinear sorption. This indicates that the analysis methods used herein to determine R and K d values for the transport data (e.g., moment analysis, frontal analysis) are robust even for systems for which transport is nonideal. This is consistent with prior theoretical and experimental studies (e.g. Glueckauf, 1955 ; Kucera, 1965 ; Buffham, 1973 , 1984 ; Villermaux, 1974 ; Valocchi, 1985 ; Brusseau, 1992 ; Brusseau et al., 2012 ).
Freundlich model parameters for the various data sets are reported in Table 2 . The Freundlich N values for PFOS sorption by the two soils are similar, 0.75 and 0.77, and are smaller than the N obtained for the sand (0.81). However, the 95% confidence intervals overlap. The N value for PFOA-sand (0.87) is larger than for PFOS-sand, although the 95% confidence intervals also overlap. The magnitude of the N values indicates a relatively moderate degree of nonlinearity.
Freundlich Model Parameters for Combined Batch and Column Sorption Isotherms
analyte | porous media | K | N |
---|---|---|---|
PFOS | Eustis | 1.07 (0.89 – 1.29) | 0.75 (0.69 – 0.82) |
PFOS | Vinton | 1.11 (0.87 – 1.41) | 0.77 (0.68 – 0.86) |
PFOS | Sand | 0.15 (0.12 – 0.19) | 0.81 (0.71 – 0.91) |
PFOA | Sand | 0.10 (0.07 – 0.14) | 0.87 (0.73 – 1.01) |
TCE | Eustis | 1.10 (0.91 – 1.33) | 0.76 (0.72 – 0.80) |
Note: the values in parentheses represent the 95% confidence intervals.
The K f values for PFOS sorption by Vinton and Eustis soils are similar, approximately 1.1. Interestingly, the organic carbon content of Eustis soil is approximately 4 times larger than that of Vinton. Brusseau et al. (2019a) hypothesized that metal oxides contributed significantly to PFOS sorption for Vinton, which has more than 3 times the metal oxide content of Eustis soil. The PFOS K f values determined for the two soils are approximately 7-times greater than the PFOS K f value for the sand, likely due to the higher organic-carbon and metal-oxide contents of the soils compared to the sand. Additionally, the K f value determined for PFOS sorption by the sand is observed to be greater than the value for PFOA sorption by the sand. This result is consistent with the molecular structures (chain length and functional group) of the two (e.g., Higgins and Luthy, 2006 ).
3.3. Sorption Isotherms: Elution-Front Integration
Isotherms determined from integration of a single elution curve for PFOS sorption by Eustis soil, Vinton soil, and sand are shown in Figures 2A , ,2B, 2B , and and2C, 2C , respectively. The isotherms determined from the integration method for Eustis and Vinton extend beyond the lower aqueous concentrations of the batch and multi-C 0 column data, while this is not the case for PFOS with sand. This reflects a degree of truncation of the elution curve for PFOS with sand. The isotherms determined from the integration method are coincident with the standard isotherms for Eustis and sand at the higher concentration range. Insufficient data points are present for the Vinton soil at the higher concentration range for comparison. However, significant deviation is observed at lower concentrations.

Isotherms for PFOS sorption from (i) integration of a single elution front, (ii) multi-C 0 column experiments, and (iii) batch experiments (the latter two are combined as “Batch & Column”). A: PFOS-Eustis soil; B: PFOS-Vinton soil; C: PFOS-Sand. The Freundlich isotherm regression is shown for combined batch and column data.
The sorbed concentrations determined with the elution-integration method are generally smaller than the standard isotherm values at lower concentrations for the Eustis and Vinton soils. Extended elution tailing was observed for PFOS transport in Eustis and Vinton soils in a previous study, which was determined to be the result primarily of rate-limited sorption/desorption ( Brusseau et al., 2019a ). The elution-integration method is based on the premise that nonlinear equilibrium sorption is the sole significant process influencing solute transport and tailing. As such, it is likely that the nonequilibrium conditions present during transport influenced application of the elution-integration method, leading to smaller calculated sorbed concentrations. This is supported by the results obtained for TCE, wherein the sorbed concentrations determined with the elution-integration method are generally smaller than the standard isotherm values (see Figure S2 ). TCE transport in the Eustis soil is influenced by rate-limited sorption/desorption to a much greater extent compared to PFOS ( Brusseau et al., 2019a ).
As noted, a significant degree of random error or noise is present at lower concentrations for the elution-integration data. This is attributed to the inherent uncertainty in concentration measurements associated with the analytical method. The noise in the isotherm can be reduced by fitting a smoothing function to the elution data, as was done for example by Bürgisser et al. (1993) . However, we did not employ such an approach so as to illustrate the noise that results from integration of the elution curve at concentrations near the analytical detection limit.
K d values were calculated from the elution-integration isotherm parameters to determine Rs for the PFOS column experiments used to generate the isotherms (see Table 1 ). The values are observed to be consistently smaller than those determined by the other methods. The discrepancies between the R values can be attributed to the smaller sorbed concentrations determined by the elution-integration method compared to those determined with the other methods, as described above.
The primary advantage of the elution-front integration method is that an entire sorption isotherm can be obtained from one column experiment. However, certain considerations should be made in using this method. Inaccurate sorbed concentrations may be obtained, particularly at lower concentrations, either because of data truncation or as a result of analytical uncertainty, as discussed above. Additionally, this method is based on the assumption that nonlinear equilibrium sorption is the sole significant process influencing transport. The accuracy of the isotherm may be compromised if dispersion, diffusive mass transfer, or nonequilibrium sorption are of significance. Prior studies have observed good agreement between adsorption isotherms determined from elution-front integration and batch experiments (Burgisser et al., 1993) and from elution-front integration and multi-C 0 column experiments ( Fesch et al., 1998 ; Metzelder et al., 2018) when equilibrium sorption conditions were ensured. However, Fesch et al. (1998) did not observe good agreement between sorption isotherms determined from elution-front integration and multiple-C 0 column experiments when the column experiments employed a pore-water velocity that was too high for equilibrium conditions to be established. The column experiments in the present study were conducted using a relatively high pore-water velocity, which likely enhanced the impact of rate-limited sorption. While this had no measurable effect on the multi- C 0 method, it apparently influenced the elution-front integration method. The use of a lower flow rate may possibly reduce the impact of sorption nonequilibrium and thereby improve the performance of the elution-front integration method.
3.4. Comparison to Literature Results
Various studies have determined Freundlich N values for the sorption of PFOS and PFOA by different soils and sediments comprising a wide range of geochemical properties. The concentration ranges for the isotherms measured in the present study span 3 to 4 orders of magnitude. Therefore, the data will be compared only to literature data with concentration ranges spanning at least two orders of magnitude or more (see Table S3 ). Higgins and Luthy (2006) determined N values between 0.81–0.96 for PFOS and 0.77–0.93 for PFOA sorption by four freshwater sediments for concentrations spanning approximately 2.5 orders of magnitude. Chen et al. (2009) determined a PFOS N value of 0.70 for one soil for concentrations spanning approximately 2.5 orders of magnitude. Guelfo and Higgins (2013) determined N values between 0.83–0.94 for PFOS and 0.89–1.08 for PFOA for three soils for a concentration range spanning approximately 3 orders of magnitude. Mejia-Avendaño et al. (2020) determined N values for PFOS between 0.73–1.08 and PFOA between 0.78–1.24 for five soils for a concentration range spanning approximately 3 orders of magnitude.
Inspection of Table S3 shows that the lower concentration range represented in the present study overlaps with the higher concentration ranges for three of the four literature studies, and that the range of those three studies extends to only approximately one log lower than the present study. In addition, the lower ranges are only slightly smaller for the Mejia-Avendaño et al. study. Overall, the N values determined in this study for PFOS and PFOA are consistent with the literature values. The soils and sediments used in the cited studies have organic carbon (OC) contents ranging from approximately 0.5% to 10%, which are significantly larger than the OC contents of the geomedia used in this study. The similarity of results obtained in the present study suggests general consistency of sorption behavior of PFOS and PFOA with these lower-OC media compared to the higher OC media typically examined to date. However, additional research is needed to substantiate this observation.
Sorption of PFOS and PFOA measured in this study for the sand can be compared to data reported in previous studies for PFAS sorption by quartz sand. Aly et al. (2018) reported K d values for PFOS and PFOA determined from batch and column experiments conducted with Ottawa quartz sand with and without the addition of two amendments aimed to enhance sorption for groundwater remediation purposes. The batch isotherms were generated for a concentration range spanning a single order of magnitude, and a linear isotherm function was fit to the data. They reported K d values of 0.075 L/kg and 0.02 L/kg for PFOS and PFOA, respectively. These values are smaller than the equivalent-K d values of 0.23 L/kg and 0.13 L/kg calculated for PFOS and PFOA, respectively, using the isotherm regression reported herein for 0.1 mg/L (the concentration employed in the Aly study). The smaller K d values reported by Aly et al. (2018) compared to this study reflect the nonlinearity determined in the present study as well as differences in the properties of the quartz sands, wherein the sand used in this study has a greater organic carbon content than the sand used by Aly et al. Guelfo et al. (2020) report Freundlich K f and N values for a suite of three PFSAs and seven PFCAs determined from batch experiments conducted with three soils and the Accusand quartz sand. Using these values, corresponding K d values of approximately 1.3 L/kg for PFOS and 0.2 L/kg for PFOA were calculated for the sand. The equivalent-K d values calculated using the isotherm regression reported herein for the same input concentrations used by Guelfo et al. (2020) are approximately 0.4 L/452 kg and 0.2 L/kg for PFOS and PFOA, respectively. The Kd values differ by a factor of ~3 for PFOS but are identical for PFOA.
K d values determined by Aly et al. (2018) from column experiments for PFOS and PFOA with the quartz sand were an order of magnitude larger than those from the batch experiments. Aly et al. (2019) conducted batch and column experiments to measure Kd values for the sorption of six PFAS by a soil. The measurements were conducted for each PFAS individually, with input concentrations of 100 μg/L used for the column experiments and an approximate one order-of-magnitude range in concentrations for the batch experiments. Mixed results were obtained with regard to consistency between column and batch data. The K d values matched for two of the six, while the column-derived K d was 2X larger for one and the batch-derived K d s were 3X larger for the remaining three PFAS. The three PFAS for which the batch K d s were larger than the respective column values represent the three with the lowest magnitudes of sorption as determined from the column experiments. The reported 95% confidence intervals did not overlap for these latter data.
Guelfo et al. (2020) conducted batch and column sorption experiments for a mixture of 10 PFAS and four porous media, with input concentrations of ~5 μg/L used for the column experiments and an approximate 1–3 orders-of-magnitude range in concentrations for the batch experiments. Retardation factors and K d values were not reported for the column experiments, so they were calculated using the same methods employed for the data presented in the current study. The column-derived K d values were then compared to equivalent-K d values determined from the reported batch K f and N values. The comparison results are mixed, with some pairs exhibiting consistent results and some not. For example, the column and batch K d s are generally similar for PFHxA. Conversely, the batch values are an average of approximately 3X larger than the column values for PFOS. Similar results were obtained using the reported measured or extrapolated batch parameters. Guelfo et al. (2020) discuss the uncertainty associated with the batch experiments and the impact on comparisons to the column data. 95% confidence intervals were calculated for the batch-derived equivalent-K d values using the data reported for soils A and B (values were not reported for soil C or the sand). The column-measured K d values for most of the PFAS-soil pairs fall within the range of the 95% confidence intervals, whereas some do not. In particular, the K d s for the longer-chain PFAS and soil B fall outside of the range. These pairs comprise the largest magnitudes of sorption for the entire set. The mixed results of the direct comparisons of batch and column K d values is consistent with the conclusion reported by Guelfo et al. (2020) that batch-derived equilibrium parameters were representative for simulating measured column BTC data for most, but not all, of the PFAS-soil pairs.
In summary, it is observed that batch and column K d values were not consistent in some cases for the three studies discussed above. Conversely, the batch and column data reported in the current study are consistent for all four PFAS-media systems tested. There are a number of possible reasons for the differences in results. The solids-solution ratio used for batch experiments in comparison to typical ratios for column systems (~4–5) has been identified as an important variable for ensuring the representativeness of batch experiments (e.g., Chang and Wang, 2002 ; Limousin et al., 2007 ; Wang et al, 2009 ). Inspection of a number of PFAS batch-sorption studies reveals that ~0.1 is a typical ratio, although some studies have used much lower values (~0.01). The solids-solution ratio used for the batch experiments in this study and that of Brusseau et al. (2019) was 0.5, and the ratios used by Aly et al. (2019) and Guelfo et al. (2020) are very similar. Hence, the solids-solution ratio does not appear to be a source of deviation. Another potential issue for batch experiments is that of agitation of the samples during the equilibration period, which may in some cases lead to particle abrasion and subsequent unrepresentative sorption measurements (e.g., Schweich et al., 1983 ). Surveying the same group of PFAS sorption studies indicates that continuous mixing is standard practice. Conversely, intermittent low-energy agitation was used for the batch experiments by Brusseau et al. (2019a) and in this study. It is also possible that uncertainties associated with the column data are influencing the comparisons. For example, concentration variability due to analytical uncertainty, such as effluent concentrations exceeding the input concentration as observed by Aly et al. (2019) and Guelfo et al. (2020) , can impact the determination of retardation factors from BTCs. Given the variable results observed for the batch-vs-column comparisons for the Aly et al. (2019) and Guelfo et al. (2020) studies, it is unclear how the use of continuous mixing, BTC uncertainty, or the impact of other experiment-condition factors, could be an issue unless they affected only select PFAS.
An aspect of interest is the comparisons between overall sorption behaviors determined by batch versus column experiments noted by the original authors for the two studies. Aly et al. (2019) observed atypical sorption behavior for PFOA in the batch experiment, wherein it exhibited greater sorption (larger K d ) than the longer-chain PFNA and PFOS. Interestingly, this atypical behavior was not observed for the column measurements; rather, the column results were fully consistent with standard behavior of K d increasing with chain length. Guelfo et al. (2013) observed atypical sorption behavior for the shortest-chain PFAS (PFBA, C3) in batch experiments, wherein the K d s for PFBA were larger than those for C4-C6 PFCAs for three soils (including soils A and B used in the 2020 study). However, Guelfo et al. (2020) note that this anomalous behavior was not observed for the column experiments. These results highlight the value of conducting an integrated set of column and batch experiments for investigating sorption processes and measuring sorption magnitudes.
4. Conclusions
The batch method has been the standard approach to date for characterizing sorption and measuring isotherms for PFAS. In contrast, miscible-displacement transport methods have received limited use, and there appears to be a perception among some researchers that column experiments are inadequate or inappropriate for characterizing sorption behavior. The results of this work demonstrate that column experiments can produce sorption data consistent with that produced by batch experiments. The magnitudes of sorption measured with the column experiments closely matched those determined with the batch experiments. This is in spite of the fact that the transport of PFOS and PFOA was influenced by rate-limited and nonlinear sorption. The consistency between the two methods indicates that the experiment and data-analysis approaches used for them were robust. These results are consistent with previous studies for other compounds that have also observed consistency between sorption isotherms determined with batch and column experiments. The excellent consistency between the two methods observed in this study does not necessarily mean that batch or column methods will always yield consistent or representative results. This will be dependent upon the use of appropriate experimental conditions.
Column experiments offer significant advantages, as they are implemented under dynamic conditions representative of transport, avoid soil abrasion that may occur during mixing during batch experiments, and involve higher soil to solution ratios that are representative of those in the subsurface environment. One major advantage is their usefulness for investigating other transport phenomena, such as sorption at fluid-fluid interfaces and soil leaching. In these cases, it is best to determine baseline sorption parameters under the same dynamic transport conditions. Column methods also have disadvantages. Compared to batch experiments, the experiment timeframe and associated workload for the column method can become impractical for systems with relatively high retention capacity. However, there are methods to overcome this constraint, including the use of cosolvent solutions (e.g., Nkedi-Kizza et al., 1987 ; Brusseau et al., 1991 ; Lee et al., 1991 ) and the application of a modified miscible-displacement column method employing small columns and high pressures termed “column chromatography” (e.g., El Guindy and Harmsen, 1989 ; Jolin et al., 2016 ; Metzelder and Schmidt, 2017 ). Another issue is that the multi-C 0 column method in particular generates substantially more samples for analysis compared to the batch method, especially when higher-resolution sampling is employed. Batch methods are correspondingly advantageous in this regard, and are especially cost- and time-effective for measuring sorption for a large number of media.
Understanding and quantifying PFAS sorption to geomedia is of critical concern for assessing the transport and fate of PFAS in the subsurface, particularly as solid-phase sorption has been shown to be an important subsurface retention mechanism. Both column and batch experiments have benefits and limitations that should be accounted for to obtain representative data. This study has demonstrated that consistent results can be obtained from both methods for sorption of PFOS and PFOA by geomedia with differing geochemical properties. The results reported herein and in prior studies ( Aly et al., 2019 ; Brusseau et al., 2019; Guelfo et al., 2020 ) highlight the value of conducting an integrated set of column and batch experiments for investigating sorption processes and measuring sorption magnitudes.
PFAS adsorption isotherms determined from column and batch methods match very well The isotherms are consistent for adsorption of PFOS and PFOA by three geomedia Retardation factors determined from column data with different methods are similar
Supplementary Material
Acknowledgements.
This research was supported by the NIEHS Superfund Research Program (grant #P42 ES 4940). The contributions of Dr. Yan were supported in part by the National Natural Science Foundation of China (NO. 41907161) and the China Postdoctoral Science Foundation (NO. 2019M662448). The authors thank the reviewers for their detailed and constructive comments.
Publisher's Disclaimer: This is a PDF file of an unedited manuscript that has been accepted for publication. As a service to our customers we are providing this early version of the manuscript. The manuscript will undergo copyediting, typesetting, and review of the resulting proof before it is published in its final form. Please note that during the production process errors may be discovered which could affect the content, and all legal disclaimers that apply to the journal pertain.
Declaration of interests
The authors declare that they have no known competing financial interests or personal relationships that could have appeared to influence the work reported in this paper.
- Allen HE; Chen YT; Li Y; Huang CP Soil partition coefficients for Cd by column desorption and comparison to batch adsorption measurements . Environ. Sci. Technol 1995, 29 , 1887–1891. [ PubMed ] [ Google Scholar ]
- Altfelder S; Streck T; Maraqa MA; Voice TC Nonequilibrium sorption of dimethylphthalate – Compatibility of batch and column techniques . Soil Sci. Soc. Am. J 2001, 65 , 102–111. [ Google Scholar ]
- Aly YH; Liu C; McInnis DP; Lyon BA; Hatton J; McCarty M; Arnold WA; Pennell KD; Simcik MF In situ remediation method for enhanced sorption of perfluoroalkyl substances onto Ottawa sand . J. Environ. Eng 2018, 144 , 04018086. [ Google Scholar ]
- Aly YH; McInnis DP; Lombardo SM; Arnold WA; Pennell KD; Hatton J; Simcik MF Enhanced adsorption of perfluoro alkyl substances for in situ remediation . Environ. Sci.: Water Res. Technol 2019, 5 , 1867–1875. [ Google Scholar ]
- Brusseau ML Nonequilibrium transport of organic chemicals: The impact of pore-water velocity . J. Contam. Hydrol 1992, 9 , 353–368. [ Google Scholar ]
- Brusseau ML; Rao PSC Sorption nonideality during organic contaminant transport in porous media . Crit. Rev. Environ. Control 1989, 19 , 33–99. [ Google Scholar ]
- Brusseau ML; Wood AL; Rao PSC Influence of organic cosolvents on the sorption kinetics of hydrophobic organic chemicals . Environ. Sci. Technol 1991, 25 , 903–910. [ Google Scholar ]
- Brusseau ML; Russo AE; Schnaar G Nonideal transport of contaminants in heterogeneous porous media : 9 – Impact of contact time on desorption and elution tailing . Chemosphere 2012, 89 , 287–292. [ PMC free article ] [ PubMed ] [ Google Scholar ]
- Brusseau ML; Khan N; Wang Y; Yan N; Van Glubt S; Carroll KC Nonideal transport and extended elution tailing of PFOS in soil . Environ. Sci. Technol 2019a, 53 , 10654–10664. [ PMC free article ] [ PubMed ] [ Google Scholar ]
- Brusseau ML; Yan N; Van Glubt S; Wang Y; Chen W; Lyu Y; Dungan B; Carroll KC; Holguin FO Comprehensive retention model for PFAS transport in subsurface systems . Wat. Res 2019b, 148 , 41–50. [ PMC free article ] [ PubMed ] [ Google Scholar ]
- Brusseau ML; Anderson RH; Guo B PFAS concentrations in soils: background levels versus contaminated sites . Science Total Environ . 2020, (in press). [ PMC free article ] [ PubMed ] [ Google Scholar ]
- Buffham BA Model-independent aspect of tracer chromatography theory . Proc. R. Soc. Lond. A 1973, 333 , 89–98. [ Google Scholar ]
- Buffham BA The velocity of chromatographic fronts or waves . J. Chromatogr. Sci 1984, 22 , 249–251. [ Google Scholar ]
- Bürgisser CS; Černík M; Borkovec M; Sticher H Determination of nonlinear adsorption isotherms from column experiments: An alternative to batch studies . Environ. Sci. Technol 1993, 27 , 943–948. [ Google Scholar ]
- Carroll KC, Artiola JF, and Brusseau ML. Transport of molybdenum in a biosolid-amended alkaline soil . Chemo . 2006, 65 , 778–785. [ PubMed ] [ Google Scholar ]
- Celorie JA; Woods SL; Vinson TS; Istok JD A comparison of sorption equilibrium distribution using batch and centrifugation methods . J. Environ. Qual 1989, 18 , 307–313. [ Google Scholar ]
- Chang TW; Wang MK Assessment of sorbent/water ratio effect on adsorption using dimensional analysis and batch experiment . Chemo . 2002, 48 , 419–426. [ PubMed ] [ Google Scholar ]
- Chen H; Chen S; Quan X; Zhao Y; Zhao H Sorption of perfluorooctane sulfonate (PFOS) on soil and oil-derived black carbon: Influence of solution pH and [Ca 2+ ] . Chemosphere 2009, 77 , 1406–1411. [ PubMed ] [ Google Scholar ]
- DeVault D The theory of chromatography . J. Am. Chem. Soc 1943, 65 , 532–540. [ Google Scholar ]
- El-Guindy S; Harmsen J Rapid column experiments to study replacement of exchangeable cations in soil samples . Netherlands J. Agric. Sci 1989, 37 ( 3 ), 213–225. 10.18174/njas.v37i3.16633 [ CrossRef ] [ Google Scholar ]
- Fesch C; Simon W; Haderlein SB; Reichert P; Schwarzenbach RP Nonlinear sorption and nonequilibrium solute transport in aggregated porous media: Experiments, process identification and modeling . J. Contam. Hydrol 1998, 31 , 373–407. [ Google Scholar ]
- Glueckauf E Theory of chromatography. Part 9. The “theoretical plate” concept in column separations.” J. Chem. Soc. Faraday Trans 1955, 51 , 34–44. [ Google Scholar ]
- Grolimund D; Borkovec M; Federer P; Sticher H Measurement of sorption isotherms with flow-through reactors . Environ. Sci. Technol 1995, 29 , 2317–2321. [ PubMed ] [ Google Scholar ]
- Guelfo JL; Higgins CP Subsurface transport potential of perfluoroalkyl acids at aqueous film-forming foam (AFFF)-impacted sites . Environ. Sci. Technol 2013, 47 , 4164–4171. [ PubMed ] [ Google Scholar ]
- Guelfo JL; Wunsch A; McCray J; Stults JF; Higgins CP Subsurface transport potential of perfluoroalkyl acids (PFAAs): Column experiments and modeling . J. Contam. Hydrol 2020, 223 , article 103661. [ PubMed ] [ Google Scholar ]
- Higgins CP; Luthy RG Sorption of perfluorinated surfactants on sediments . Environ. Sci. Technol 2006, 40 , 7251–7256. [ PubMed ] [ Google Scholar ]
- Jolin WC; Sullivan J; Vasudevan D; MacKay AA Column chromatography to obtain organic cation sorption isotherms . Environ. Sci. Technol 2016, 50 , 8196–8204. [ PubMed ] [ Google Scholar ]
- Kempf A; Brusseau ML Impact of non-ideal sorption on low-concentration tailing behavior for atrazine transport in two natural porous media . Chemosphere 2009, 77 , 877–882. [ PMC free article ] [ PubMed ] [ Google Scholar ]
- Kucera E Contribution to the theory of chromatography linear non-equilibrium elution chromatography . J. Chromatogr 1965, 19 , 237–248. [ PubMed ] [ Google Scholar ]
- Lee LS; Rao PSC; Brusseau ML; Ogwada RA Nonequilibrium sorption of organic contaminants during flow through columns of aquifer materials . Environ. Toxicol. Chem 1988, 7 , 779–793. [ Google Scholar ]
- Lee LS; Rao PSC; Brusseau ML Nonequilibrium sorption and transport of neutral and ionized chlorophenols . Environ. Sci. Technol 1991, 25 , 722–729. [ Google Scholar ]
- Limousin G; Gaudet JP; Charlet L; Szenknect S; Barthès V; Krimissa M Sorption isotherms: A review on physical bases, modeling and measurement . Appl. Geochem 2007, 22 , 249–275. [ Google Scholar ]
- Lv X; Sun Y; Ji R; Gao B; Wu J; Lu Q; Jiang H Physiochemical factors controlling the retention and transport of perfluorooctanoic acid (PFOA) in saturated sand and limestone porous media . Wat. Res 2018, 141 , 251–258. [ PubMed ] [ Google Scholar ]
- Lyu Y; Brusseau ML; Chen W; Yan N; Fu X; Lin X Adsorption of PFOA at the air-water interface during transport in unsaturated porous media . Environ. Sci. Technol 2018, 52 , 7745–7753. [ PMC free article ] [ PubMed ] [ Google Scholar ]
- Lyu X; Liu X; Sun Y; Ji R; Gao B; Wu J Transport and retention of perfluorooctanoic acid (PFOA) in natural soils: Importance of soil organic matter and mineral contents, and solution ionic strength . J. Contam. Hydrol 225 , article 103477. [ PubMed ] [ Google Scholar ]
- Lyu Y; Brusseau ML The influence of solution chemistry on air-water interfacial adsorption and transport of PFOA in unsaturated porous media . Sci. Total Environ 2020, 713 , article 136744. [ PMC free article ] [ PubMed ] [ Google Scholar ]
- MacIntyre WG; Stauffer TB; Antworth CP A comparison of sorption coefficients determined by batch, column, and box methods on a low organic carbon aquifer material . Groundwater 1991, 29 , 908–913. [ Google Scholar ]
- Maraqa MA; Zhao X; Wallace RB; Voice TC Retardation coefficients of nonionic organic compounds determined by batch and column techniques . Soil Sci. Soc. Am. J 1998, 62 , 142–152. [ Google Scholar ]
- Mayer SW; Tompkins ER Ion exchange as a separations method. IV. A theoretical analysis of the column separations process . J. Am. Chem. Soc 1947, 69 2866–2874. [ PubMed ] [ Google Scholar ]
- McKenzie ER; Siegrist RL; McCray JE; Higgins CP Effects of chemical oxidants on perfluoroalkyl acid transport in one-dimensional porous media columns . Environ. Sci. Technol 2015, 49 , 1681–1689. [ PubMed ] [ Google Scholar ]
- Mejia-Avendaño S; Zhi Y; Yan B; Liu J Sorption of polyfluoroalkyl surfactants on surface soils: Effect of molecular structures, soil properties, and solution chemistry . Environ. Sci. Technol 2020, 54 , 1513–1521. [ PubMed ] [ Google Scholar ]
- Metzelder F; Schmidt TC Environmental conditions influencing sorption of inorganic anions to multiwalled carbon nanotubes studied by column chromatography . Environ. Sci. Technol 2017, 51 , 4928–4935. [ PubMed ] [ Google Scholar ]
- Mon J; Flury M; Harsh JB Sorption of four triarylmethane dyes in a sandy soil determined by batch and column experiments . Geoderma 2006, 133 , 217–224. [ Google Scholar ]
- Nkedi-Kizza P; Rao PSC; Hornsby AG Influence of organic cosolvents on leaching of hydrophobic organic chemicals through soils . Environ. Sci. Technol 1987, 21 , 1107–1111. [ Google Scholar ]
- Schnaar G; Brusseau ML Nonideal transport of contaminants in heterogeneous porous media: 11. Testing the experiment condition dependency for sorption-desorption . Water Air Soil Pollut . 2014, 225 article 2136. [ PMC free article ] [ PubMed ] [ Google Scholar ]
- Schneider P; Smith JM Adsorption rate constants from chromatography . AIChE 1968, 14 , 762–771. [ Google Scholar ]
- Schultz MM; Barofsky DF; Field JA, 2004. Quantitative determination of fluorotelomer sulfonates in groundwater by LC MS/MS . Environ. Sci. Technol 2004, 38 , 1828e1835 10.1021/es035031j. [ PubMed ] [ CrossRef ] [ Google Scholar ]
- Schweich D; Sardin M; Gaudet JP Measurement of a cation exchange isotherm from elution curves obtained in a soil column: Preliminary results . Soil Sci. Soc. Am. J 1983, 47 , 32–37. [ Google Scholar ]
- Valocchi AJ Validity of the local equilibrium assumption for modeling sorbing solute transport through homogeneous soil . Water Resour. Res 1985, 21 , 808–820. [ Google Scholar ]
- Vierke L; Möller A; Klitzke S Transport of perfluoroalkyl acids in a water-saturated sediment column investigated under near-natural conditions . Environ. Pollut 2014, 186 , 7–13. [ PubMed ] [ Google Scholar ]
- Villermaux J Determination of chromatographic peaks under the influence of mass transfer phenomena . J. Chromatogr. Sci 1974, 12 , 822–831. [ Google Scholar ]
- Wang TH; Li MH; Teng SP Bridging the gap between batch and column experiments: A case study of Cs adsorption on granite . J. Hazard Mater 2009, 161 , 409–415. [ PubMed ] [ Google Scholar ]
- Yan N, Ji Y, Zhang B, Zheng X, and Brusseau ML. Transport of GenX in saturated and unsaturated porous media . Environ. Sci. Technol 2020, 54 , 11876–11885. [ PMC free article ] [ PubMed ] [ Google Scholar ]
Information
- Author Services
Initiatives
You are accessing a machine-readable page. In order to be human-readable, please install an RSS reader.
All articles published by MDPI are made immediately available worldwide under an open access license. No special permission is required to reuse all or part of the article published by MDPI, including figures and tables. For articles published under an open access Creative Common CC BY license, any part of the article may be reused without permission provided that the original article is clearly cited. For more information, please refer to https://www.mdpi.com/openaccess .
Feature papers represent the most advanced research with significant potential for high impact in the field. A Feature Paper should be a substantial original Article that involves several techniques or approaches, provides an outlook for future research directions and describes possible research applications.
Feature papers are submitted upon individual invitation or recommendation by the scientific editors and must receive positive feedback from the reviewers.
Editor’s Choice articles are based on recommendations by the scientific editors of MDPI journals from around the world. Editors select a small number of articles recently published in the journal that they believe will be particularly interesting to readers, or important in the respective research area. The aim is to provide a snapshot of some of the most exciting work published in the various research areas of the journal.
Original Submission Date Received: .
- Active Journals
- Find a Journal
- Journal Proposal
- Proceedings Series
- For Authors
- For Reviewers
- For Editors
- For Librarians
- For Publishers
- For Societies
- For Conference Organizers
- Open Access Policy
- Institutional Open Access Program
- Special Issues Guidelines
- Editorial Process
- Research and Publication Ethics
- Article Processing Charges
- Testimonials
- Preprints.org
- SciProfiles
- Encyclopedia
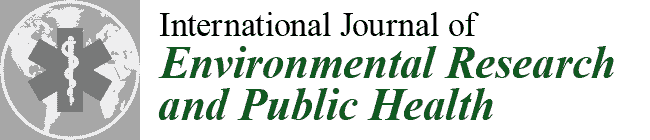
Article Menu

- Subscribe SciFeed
- Recommended Articles
- PubMed/Medline
- Google Scholar
- on Google Scholar
- Table of Contents
Find support for a specific problem in the support section of our website.
Please let us know what you think of our products and services.
Visit our dedicated information section to learn more about MDPI.
JSmol Viewer
Soil column experimental study on the effect of soil structure disturbance on water chemistry.
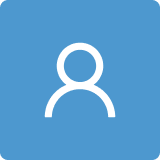
1. Introduction
2. materials and methods, 2.1. mineral composition analysis, 2.2. soil and groundwater in natural conditions, 2.3. the soil column experiment, 2.4. sample analyses, 3.1. mineral composition of the soil, 3.2. so 4 in soil and groundwater under the natural conditions, 3.3. the soil column experiment, 4. discussion, 4.1. so 4 -increasing process in effluent water, 4.2. soil so 4 -containing evaporites dissolution mechanism, 4.3. implications for chemical compositions, 5. conclusions, author contributions, institutional review board statement, informed consent statement, data availability statement, acknowledgments, conflicts of interest.
- Li, Z.Q.; Qi, Z.Y.; Qi, S.W.; Zhang, L.X.; Hou, X.K. Microstructural changes and micro–macro-relationships of an intact, compacted and remolded loess for land-creation project from the Loess Plateau. Environ. Earth Sci. 2021 , 80 , 593. [ Google Scholar ] [ CrossRef ]
- Zhang, L.X.; Qi, S.W.; Ma, L.N.; Guo, S.F.; Li, Z.Q.; Li, G.L.; Yang, J.J.; Zou, Y.; Li, T.L.; Hou, X.K. Three-dimensional pore characterization of intact loess and compacted loess with micron scale computed tomography and mercury intrusion porosimetry. Sci. Rep. 2020 , 10 , 8511. [ Google Scholar ] [ CrossRef ] [ PubMed ]
- Bouma, J. Influence of soil macroporosity on environmental quality. Adv. Agron 1991 , 46 , 1–37. [ Google Scholar ]
- Kumar, P.; Mahajan, A.K.; Kumar, A. Groundwater geochemical facie: Implications of rock-water interaction at the Chamba city (H.P.), northwest Himalaya, India. Environ. Sci. Pollut. Res. 2020 , 27 , 9012–9026. [ Google Scholar ] [ CrossRef ]
- Speight, J.G. Water Chemistry. In Natural Water Remediation: Chemistry and Technology ; Speight, J.G., Ed.; Butterworth-Heinemann: Boston, MA, USA, 2020; pp. 91–129. [ Google Scholar ] [ CrossRef ]
- Jin, Z.D.; West, A.J.; Zhang, F.; An, Z.S.; Hiton, R.G.; Yu, J.M.; Wang, J.; Li, G.; Deng, L.; Wang, X.L. Seismically enhanced solute fluxes in the Yangtze River headwaters following the A.D. 2008 Wenchuan earthquake. Geology 2016 , 44 , 47–50. [ Google Scholar ] [ CrossRef ] [ Green Version ]
- White, A.F.; Brantley, S.L. The effect of time on the weathering of silicate minerals: Why do weathering rates differ in the laboratory and field? Chem. Geol. 2003 , 202 , 479–506. [ Google Scholar ] [ CrossRef ]
- Karikari-Yeboah, O.; Addai-Mensah, J. Assessing the impact of preload on pyrite-rich sediment and groundwater quality. Environ. Monit. Assess. 2017 , 189 , 58. [ Google Scholar ] [ CrossRef ]
- Wang, J.; Jin, Z.D.; Hilton, R.G.; Zhang, F.; Densmore, A.L.; Li, G.; West, A.J. Controls on fluvial evacuation of sediment from earthquaketriggered landslides. Geology 2015 , 43 , 115–118. [ Google Scholar ] [ CrossRef ] [ Green Version ]
- Emberson, R.; Hovius, N.; Galy, A.; Marc, O. Chemical weathering in active mountain belts controlled by stochastic bedrock landsliding. Nat. Geosci. 2016 , 9 , 42–45. [ Google Scholar ] [ CrossRef ]
- Huang, T.M.; Li, Z.B.; Long, Y.; Zhang, F.; Pang, Z.H. Role of desorption-adsorption and ion exchange in isotopic and chemical (Li, B, and Sr) evolution of water following water–rock interaction. J. Hydrol. 2022 , 610 , 127800. [ Google Scholar ] [ CrossRef ]
- Claesson, L.; Skelton, A.; Graham, C.; Dietl, C.; Mörth, M.; Torssander, P.; Kockum, I. Hydrogeochemical changes before and after a major earthquake. Geology 2004 , 32 , 641–644. [ Google Scholar ] [ CrossRef ]
- Skelton, A.; Andrén, M.; Kristmannsdóttir, H.; Stockmann, G.; Mörth, C.-M.; Sveinbjörnsdóttir, Á.; Jónsson, S.; Sturkell, E.; Guðrúnardóttir, H.R.; Hjartarson, H.; et al. Changes in groundwater chemistry before two consecutive earthquakes in Iceland. Nat. Geosci. 2014 , 7 , 752–756. [ Google Scholar ] [ CrossRef ] [ Green Version ]
- Zhang, L.X.; Qi, S.W.; Yu, Y.T.; Zhang, Y.G.; Li, Z.Q.; Hou, X.K.; Ma, L.N.; Zou, Y.; Guo, S.F.; Peng, J.B. A comparative study on the physical properties of natural sedimentary loess and manual filling compacted loess. Environ. Earth Sci. 2021 , 80 , 721. [ Google Scholar ] [ CrossRef ]
- Liu, T.-S. Material Composition and Structure of Loess ; Science Press: Beijing, China, 1966. [ Google Scholar ]
- Freese, K.; Jambor, K.; Abbas, A.R.; Senko, J.M.; Cutright, T.J. Origin and formation of sulfate in soils from three ohio counties. Geotech. Geol. Eng. 2016 , 34 , 1327–1343. [ Google Scholar ] [ CrossRef ]
- Gamboa, C.; Godfrey, L.; Herrera, C.; Custodio, E.; Soler, A. The origin of solutes in groundwater in a hyper-arid environment: A chemical and multi-isotope approach in the Atacama Desert, Chile. Sci. Total Environ. 2019 , 690 , 329–351. [ Google Scholar ] [ CrossRef ] [ PubMed ]
- Huang, T.M.; Ma, B.Q. The origin of major ions of groundwater in a loess aquifer. Water 2019 , 11 , 2464. [ Google Scholar ] [ CrossRef ] [ Green Version ]
- Langmuir, D. Aqueous Environmental Geochemistry ; Prentice Hall: Upper Saddle River, NJ, USA, 1997. [ Google Scholar ]
- Li, P.Y.; He, S.; Yang, N.N.; Xiang, G. Groundwater quality assessment for domestic and agricultural purposes in Yan’an City, northwest China, implications to sustainable groundwater quality management on the Loess Plateau. Environ. Earth Sci. 2018 , 77 , 775. [ Google Scholar ] [ CrossRef ]
- Long, Y.; Huang, T.M.; Zhang, F.; Li, Z.B.; Ma, B.Q.; Li, Y.M.; Pang, Z.H. Origin of sulphate in the unsaturated zone and groundwater of a loess aquifer. Hydrol. Process. 2021 , 35 , e14166. [ Google Scholar ] [ CrossRef ]
- Qian, H.; Ma, Z.Y.; Li, P.Y. Hydrogeochemistry ; Geological Publishing House: Beijing, China, 2005. [ Google Scholar ]
- Toscani, L.; Venturelli, G.; Boschetti, T. Sulphide-bearing waters in Northern Apennines, Italy: General features and water-rock interaction. Aquat. Geochem. 2001 , 7 , 195–216. [ Google Scholar ] [ CrossRef ]
- Amin, M.G.M.; Pedersen, C.Ø.; Forslund, A.; Veith, T.L.; Laegdsmand, M. Influence of soil structure on contaminant leaching from injected slurry. J. Environ. Manag. 2016 , 184 , 289–296. [ Google Scholar ] [ CrossRef ]
- Griessbach, E.F.C.; Copin, A.; Deleu, R.; Dreze, P. Mobility of a siliconepolyether studied by leaching experiments through disturbed and undisturbed soil columns. Sci. Total Environ. 1998 , 221 , 159–169. [ Google Scholar ] [ CrossRef ]
- Rodríguez-Oroz, D.; Lasheras, E.; Aldabe, J.; Elustondo, D.; Santamaría, J.M.; Garrigó, J. Heavy metals mobility in experimental disturbed and undisturbed acid soil columns in Spanish Pyrenees. Environ. Eng. Manag. J. 2012 , 11 , 1149–1158. [ Google Scholar ] [ CrossRef ]
- Huang, T.M.; Li, Z.B.; Mayer, B.; Nightingale, M.; Li, X.; Li, G.F.; Long, Y.; Pang, Z.H. Identification of geochemical processes during hydraulic fracturing of a shale gas reservoir: A controlled field and laboratory water-rock interaction experiment. Geophys. Res. Lett. 2020 , 47 , e2020GL090420. [ Google Scholar ] [ CrossRef ]
- Wang, J.D.; Li, P.; Gu, Q.; Xu, Y.J.; Gu, T.F. Changes in tensile strength and microstructure of loess due to vibration. J. Asian Earth Sci. 2019 , 169 , 298–307. [ Google Scholar ] [ CrossRef ]
- Huang, T.M.; Ma, B.Q.; Pang, Z.H.; Li, Z.; Li, Z.B.; Long, Y. How does precipitation recharge groundwater in loess aquifers? Evidence from multiple environmental tracers. J. Hydrol. 2020 , 583 , 124532. [ Google Scholar ] [ CrossRef ]
- Krouse, H.R.; Mayer, B. Sulphur and Oxygen Isotopes in Sulphate. In Environmental Tracers in Subsurface Hydrology ; Cook, P.G., Herczeg, A.L., Eds.; Kluwer Academic Publishers: Boston, MA, USA, 2000; pp. 195–231. [ Google Scholar ] [ CrossRef ]
- Zhen, W.W.; Lv, H.M.; Cui, K.; Wu, G.P. Influence on strength of site soils about chlorine and sulfate salt in dry environment. J. Eng. Geol. 2014 , 22 , 333–338. (In Chinese) [ Google Scholar ] [ CrossRef ]
- Wei, Y.Z.; Yao, Z.H.; Chong, X.L.; Zhang, J.H.; Zhang, J. Microstructure of unsaturated loess and its influence on strength characteristics. Sci. Rep. 2022 , 12 , 1502. [ Google Scholar ] [ CrossRef ]
- Bernadiner, M.G. A capillary microstructure of the wetting front. Transp. Porous Media 1998 , 30 , 251–265. [ Google Scholar ] [ CrossRef ]
- Wu, Z.; Zhang, H. Experimental study on entrapped air content in quasi-saturated soil subjected to steady ponded water infiltration. Chin. J. Geotech. Eng. 2012 , 34 , 274–279. (In Chinese) [ Google Scholar ]
- Lewis, J.; Sjostrom, J. Optimizing the experimental design of soil columns in saturated and unsaturated transport experiments. J. Contam. Hydrol. 2010 , 115 , 1–13. [ Google Scholar ] [ CrossRef ]
Click here to enlarge figure
Sample | Depth (m) | Quartz | Potassium Feldspar | Plagioclase | Calcite | Dolomite | Clay | Mineral Composition for Clay | |
---|---|---|---|---|---|---|---|---|---|
S1 | 9.75–10 | 43.0 | 3.4 | 16.5 | 9.1 | 0 | 28.0 | Smectite | 0 |
Illite-smectite | 32 | ||||||||
Illite | 49 | ||||||||
Kaolinite | 5 | ||||||||
Chlorite | 14 | ||||||||
S2 | 29.5–30 | 44.7 | 2.9 | 11.8 | 13.6 | 0 | 27.0 | Smectite | 0 |
Illite-smectite | 20 | ||||||||
Illite | 60 | ||||||||
Kaolinite | 7 | ||||||||
Chlorite | 13 | ||||||||
S3 | 54.5–55 | 44.5 | 4.3 | 15.3 | 16.4 | 3.4 | 16.1 | Smectite | 0 |
Illite-smectite | 21 | ||||||||
Illite | 58 | ||||||||
Kaolinite | 9 | ||||||||
Chlorite | 12 |
Type | Sample No. | Time | Volume | SO | Cl | SO /Cl | δ S-SO | δ O-SO |
---|---|---|---|---|---|---|---|---|
(h) | (L) | (mg/L) | (mg/L) | (‰) | (‰) | |||
Influent water | G0 | 40.1 | 6.8 | 5.5 | 1.2 | 7.2 | −2.3 | |
SO -containing evaporites endmember | L0 | 14.1 | 9.1 | |||||
Effluent water | L1 | 9 | 0.98 | 96.6 | 11.2 | 8.6 | 14.2 | 5.9 |
L2 | 29 | 2.02 | 8.2 | 7.1 | 1.2 | 9.4 | 0.8 | |
L3 | 50 | 1.97 | 7.2 | 5.8 | 1.2 | 7.7 | −1.2 | |
L4 | 74 | 2.26 | 6.9 | 5.1 | 1.4 | 8.1 | −0.9 | |
L5 | 106 | 3.14 | 7.5 | 5.2 | 1.4 | 7.3 | −1.6 | |
L6 | 160 | 5.23 | 6.0 | 5.1 | 1.2 | 7.9 | −0.3 | |
L7 | 264 | 9.28 | 6.4 | 5.3 | 1.2 | 7.6 | −2.0 | |
L8 | 329 | 6.15 | 6.6 | 5.0 | 1.3 | 8.0 | −1.7 | |
L9 | 425 | 8.21 | 6.6 | 5.7 | 1.2 | 8.0 | −1.9 | |
Remaining water-extractable soil water | R1 | 8.9 | 3.6 | 2.5 | * | * |
MDPI stays neutral with regard to jurisdictional claims in published maps and institutional affiliations. |
Share and Cite
Long, Y.; Huang, T.; Zhang, F.; Zhao, Y. Soil Column Experimental Study on the Effect of Soil Structure Disturbance on Water Chemistry. Int. J. Environ. Res. Public Health 2022 , 19 , 15673. https://doi.org/10.3390/ijerph192315673
Long Y, Huang T, Zhang F, Zhao Y. Soil Column Experimental Study on the Effect of Soil Structure Disturbance on Water Chemistry. International Journal of Environmental Research and Public Health . 2022; 19(23):15673. https://doi.org/10.3390/ijerph192315673
Long, Yin, Tianming Huang, Fen Zhang, and Yajing Zhao. 2022. "Soil Column Experimental Study on the Effect of Soil Structure Disturbance on Water Chemistry" International Journal of Environmental Research and Public Health 19, no. 23: 15673. https://doi.org/10.3390/ijerph192315673
Article Metrics
Article access statistics, further information, mdpi initiatives, follow mdpi.
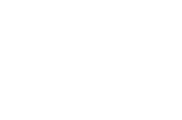
Subscribe to receive issue release notifications and newsletters from MDPI journals
- Analytical Chemistry
Column Chromatography
What is column chromatography.
In chemistry, Column chromatography is a technique which is used to separate a single chemical compound from a mixture dissolved in a fluid.
Column chromatography separates substances based on differential adsorption of compounds to the adsorbent as the compounds move through the column at different rates which allows them to get separated in fractions. This technique can be used on a small scale as well as large scale to purify materials that can be used in future experiments. This method is a type of adsorption chromatography technique.
Table of Content
- Applications
- Frequently Asked Questions – FAQs
Column Chromatography Principle
When the mobile phase along with the mixture that needs to be separated is introduced from the top of the column, the movement of the individual components of the mixture is at different rates. The components with lower adsorption and affinity to the stationary phase travel faster when compared to the greater adsorption and affinity with the stationary phase. The components that move fast are removed first whereas the components that move slowly are eluted out last.
The adsorption of solute molecules to the column occurs in a reversible manner. The rate of the movement of the components is expressed as:
R f = the distance travelled by solute/ the distance travelled by the solvent
R f is the retardation factor.
Column Chromatography Diagram
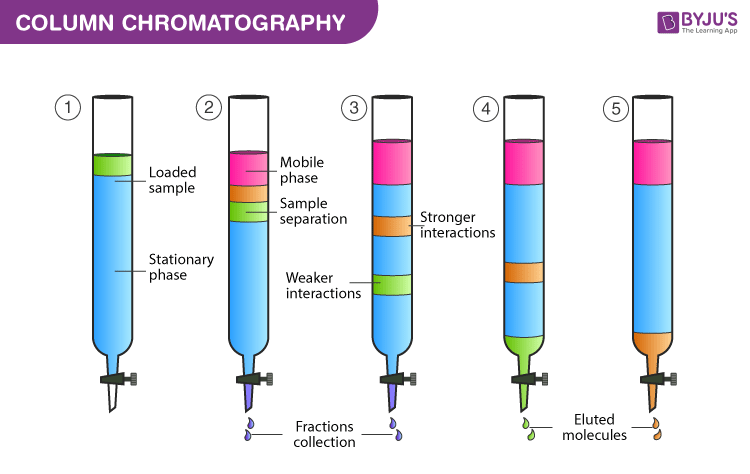
Elution is a chemical process that involves removing a material’s ions by ion exchange with another material. The chromatographic technique of extracting an adsorbed substance from a solid adsorbing media using a solvent. The eluent is the solvent or mobile phase that passes through the column. When the polarity of the eluent matches the polarity of the molecules in the sample, the molecules desorb from the adsorbent and dissolve in the eluent.
The fraction of the mobile phase that transports the sample components is known as eluent. The mixture of solute and solvent that exits the column is known as an eluate. The eluate is made up of the mobile phase and analytes. A substance that separates and moves constituents of a mixture through the column of a chromatograph. The eluent in liquid chromatography is a liquid solvent whereas in gas chromatography is a carrier gas.
Column Chromatography Procedure
Before starting with the Column Chromatography Experiment let us understand the different phases involved.
Mobile phase – This phase is made up of solvents and it performs the following functions:
- It acts as a solvent-sample mixture that can be introduced in the column.
- It acts as a developing agent – helps in the separation of components in the sample to form bands.
- It acts as an eluting agent – the components that are separated during the experiment are removed from the column
- Some examples of solvents used as mobile phases based on their polarity are – ethanol, acetone, water, acetic acid , pyridine, etc.
Stationary phase – It is a solid material which should have good adsorption properties and meet the conditions given below:
- Shape and size of particle: Particles should have a uniform shape and size in the range of 60 – 200μ in diameter.
- Stability and inertness of particles: high mechanical stability and chemically inert. Also, no reaction with acids or bases or any other solvents was used during the experiment.
- It should be colourless, inexpensive and readily available.
- Should allow free flow of mobile phase
- It should be suitable for the separation of mixtures of various compounds.
Column Chromatography Experiment
- The stationary phase is made wet with the help of solvent as the upper level of the mobile phase and the stationary phase should match. The mobile phase or eluent is either solvent or a mixture of solvents. In the first step the compound mixture that needs to be separated, is added from the top of the column without disturbing the top level. The tap is turned on and the adsorption process on the surface of silica begins.
- Without disturbing the stationary phase solvent mixture is added slowly by touching the sides of the glass column. The solvent is added throughout the experiment as per the requirement.
- The tap is turned on to initiate the movement of compounds in the mixture. The movement is based on the polarity of molecules in the sample. The non-polar components move at a greater speed when compared to the polar components.
- For example, a compound mixture consists of three different compounds viz red, blue, green then their order based on polarity will be as follows blue>red>green
- As the polarity of the green compound is less, it will move first. When it arrives at the end of the column it is collected in a clean test tube. After this, the red compound is collected and at last blue compound is collected. All these are collected in separate test tubes.
Column Chromatography Applications
- Column Chromatography is used to isolate active ingredients.
- It is very helpful in separating compound mixtures.
- It is used to determine drug estimation from drug formulations.
- It is used to remove impurities.
- Used to isolate metabolites from biological fluids.
Types of Column Chromatography:
1. Adsorption column chromatography – Adsorption chromatography is a technique of separation, in which the components of the mixture are adsorbed on the surface of the adsorbent.
2. Partition column chromatography – The stationary phase, as well as mobile phase, are liquid in partition chromatography .
3. Gel column chromatography – In this method of chromatography, the separation takes place through a column packed with gel. The stationary phase is a solvent held in the gap of a solvent.
Frequently Asked Questions – FAQs
What is the principle involved in column chromatography.
The basic principle involved in column chromatography is to adsorb solutes of the solution with the help of a stationary phase and further separate the mixture into discrete components.
What is column chromatography?
It is a precursory technique used in the purification of compounds based on their hydrophobicity or polarity. In this chromatography process, the molecule mixture is separated depending on its differentials partitioning between a stationary phase and a mobile phase.

What is the main advantage of column chromatography?
The main advantage of this chromatography technique is that the stationary phase is less expensive and can be easily disposed of as it undergoes recycling.
How are the compounds separated in this technique?
The separation is similar to that of TLC where the compound mixture is carried by a mobile phase via a stationary phase.
Which compounds elute out first in the column chromatography technique?
Non-polar compounds. The polar compounds will strongly commune with the silica when compared to the non-polar compounds.
What is elution in column chromatography?
What are the limitations of column chromatography, what are the different types of column chromatography, what is an adsorption column chromatography, what is gel column chromatography.
To learn more about the different types of Column Chromatography from the experts, register to BYJU’S now!
Other important links:
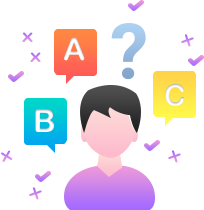
Put your understanding of this concept to test by answering a few MCQs. Click ‘Start Quiz’ to begin!
Select the correct answer and click on the “Finish” button Check your score and answers at the end of the quiz
Visit BYJU’S for all Chemistry related queries and study materials
Your result is as below
Request OTP on Voice Call
CHEMISTRY Related Links | |
Leave a Comment Cancel reply
Your Mobile number and Email id will not be published. Required fields are marked *
Post My Comment

What is developing agent?
Chromatographic procedure generally involves introducing at the top of the column the mixture of the components to be separated, developing the mixture with a suitable agent, and collecting the components in separate effluent fractions. The best developing agent in thin-layer chromatography was Petroleum ether: ethyl acetate.

Register with BYJU'S & Download Free PDFs
Register with byju's & watch live videos.

OR WAIT null SECS
- Editorial Information
- Do Not Sell My Personal Information
- Privacy Policy
- Terms and Conditions

© 2024 MJH Life Sciences ™ and BioPharm International . All rights reserved.
Efficiency Measurements for Chromatography Columns
Misinterpreting the effluent profiles obtained during tracer measurements performed for determining packing quality can often lead to excessively large percolation velocities and exaggeration of packing problems. Highly useful and reliable information can be obtained through characterization of tracer effluent curves using the method of moments, information that could be critical for successful scale-up of chromatographic steps. This is the sixth in the "Elements of Biopharmaceutical Production" series.
Anurag Rathore
PLATE THEORY
The plate theory of chromatography was introduced by Martin and Synge in 1941. 1 It offered the first description of the development of quasi-Gaussian bands in linear elution chromatography, which is defined as conditions where the solute is partitioned between the mobile phase and stationary phase in the presence of a linear isotherm. Plate theory describes a column as consisting of a number of theoretical plates such that at each plate, the solute is equilibrated between two phases. It is assumed that the band spreading due to diffusion of solute from one plate to another is negligibly small, and that at equilibrium the partition coefficient of a solute is independent of its concentration and of the presence of other solutes. It was observed that after a certain length of time, the zone profile became Gaussian in shape.
Martin and Synge defined a height equivalent to a theoretical plate (HETP) as "the thickness of a layer in the column such that the eluting mobile phase is in equilibrium with the solute concentration in the stationary phase." They argued that the HETP is a constant through a given column, except when the ratio of the mobile phase concentrations at the entrance and exit differ greatly from unity. The plate height depends on the solute diffusivity in the mobile phase, and is directly proportional to the flowrate ( v 0 ) and the square of the particle diameter ( d p 2 ). Though marred by several inconsistencies, 2,3 the originality and simplicity of the plate model made it very popular.
In 1955, Giddings and Eyring employed probability (stochastic) concepts to describe the differential migration process of chromatography. 2 They developed a rigorous probability theory that gave a precise description of the influence of simple adsorption-desorption processes on the zone profile. Their approach later led to the simplified and approximate theory of zone dispersion, often referred to as the random walk theory of chromatography, 3 for the situation when the time spent by the solutes inside the column is large enough to allow each molecule to undergo a large number of individual sorption and desorption steps.
Let us delve into plate theory and the normal probability, or Gaussian, distribution upon which it is based. 1 Equation (1) describes the Gaussian distribution and Equation (2) the time elapsed. All symbols are defined in a sidebar.
We plotted two examples in Figure 1. The graphical interpretation of s is the half-width of the distribution at its inflection point. If the curves in this figure represent effluent concentrations from a tracer experiment on a chromatographic column, the number of plates is defined as:
Figure 1. A Gaussian Distribution
and the height of a plate, H is:
The vertical scale, corresponding to effluent concentration in our situation, does not affect calculation of H or N .
When time is expressed as in Figure 1, the value of s is independent of both the mass of pulse fed (if the effluent curve is Gaussian) and the column diameter. Moreover the mean residence time equals the time at which the maximum concentration, t max , occurs. The number of plates is expressed in Equation (5).
The Short-cut Method and the Péclet Number
If effluent curves were always Gaussian, then calculating plate numbers and heights would be a simple process. There would, of course, be the nagging problem of determining standard deviations from experimental data, and it has been a common practice to use short-cut methods for this purpose. We discuss a set recommended by the American Society for Testing and Materials. 4 Equation (6) from the ASTM is a variant of Equation (5).
If the peak is Gaussian, then the standard deviation is the half-width at 60.7% of peak height and it reduces to Equation (7).
Unfortunately no chromatographic peaks are truly Gaussian, and this is the problem we must deal with next. Figure 2 shows simulated solute concentrations along a column using L as the distance along the column from inlet. 5 It makes evident that solute profiles within a column are functions of percolation velocity, adsorbent particle diameters and solute diffusivity as expressed via a dimensionless group known as the Péclet number.
Both peaks are left-skewed , but the magnitude of skewness increases with Pé , or inversely with diffusivity. The primary reason for this is intra-particle diffusional transients, and the degree of skewness depends primarily on a ratio of time constants as in Equation (9).
Equation (10) shows that k is always in the range 0 to 1.
The same value of K D is used for both curves of Figure 2, so we can extract some useful information. First, we note that skewness becomes increasingly severe as T D /T v becomes large relative to the time required for solute to pass a particle. Next we see that the definitions of N and H are ambiguous, and no single measure is sufficient. Moreover the mean residence time is no longer the same as that for appearance of the concentration peak.
Figure 2. Tracer Distortion-simulation
A low-molecular weight tracer is commonly used to characterize a chromatographic column. Frequently, a low-molecular weight tracer will show less skewness that a larger solute. This is shown in Figure 3 for actual effluent curves. The acetone tracer has a sharper peak than for the desired product, a globular protein. Even the acetone tracer distribution is appreciably skewed, and this skewness shows a fundamental difference between the effluent curves of this figure and the intra-column profiles of Figure 2. The later bits of acetone to appear have been in the column longer and have therefore had more opportunity to disperse. In summary, no effluent curves are truly Gaussian, and the plate concept as defined earlier represents an ideal case that can only be approached as a limit for very large N .
Figure 3. Tracer Distortion - Effect of Experimental Conditions Column: 1.6 Ã 8.8 cm ToyoPearl SP 650M. Linear velocity: 200 cm/hr. Mobile Phase: 150mM Citrate, Solute Pulses: 500 mL of 10 mg/mL globular protein, 500 mL of 5% acetone.
Measures of Column Efficiency
There is no simple way to resolve this ambiguity, but it is clear that we now need at least two measures of tracer shape — one for the extent of dispersion about the mean residence time and another for skewness. In our experience, these have generally proved sufficient, and we recommend the use of statistical moments.These moments are described in Equations (11), (12), and (13).
The zeroeth moment is the area under the effluent curve, which is effectively the mass of the solute pulse fed. The first moment is equal to the mean residence time. M 2 is the second moment of concentration with respect to time about the mean residence time. It describes the breadth of the peak and can be calculated from the chromatogram and used to estimate the variance of Gaussian or near-Gaussian peaks. The first and the second moments of a peak are independent of the amount of the component as they are normalized to the peak area. It should be noted that Equations (11) thru (13) provide definitions for moments in the temporal time frame, whereas peak analysis can be performed in either the temporal or spatial timeframe. These moments can be related through the migration velocity, u .
While the peak traverses the column, its variance increases due to longitudinal diffusion and other dispersion effects. 6 The variance of the peak is an additive quantity. That means the total variance can be expressed as the sum of variances coming from all the contributors to dispersion. 7,8
To characterize skewness we suggest using the third moment about the mean residence time according to Equation (14).
Skewness also can be characterized by differences between the right- and left-hand half widths, but there is a major advantage to using moments throughout. This is because M 1 , M 2 , and M 3 are additive. 9 The observed values of these three moments are just the sums of those for the column itself and those for all of the auxiliary apparatus — tubing, headers, and even detectors. This is particularly important during scale-up as auxiliary apparatus can have major effects on effluent curves.
Figure 4. Comparison of Techniques to Characterize Column Effluent Curves Experimental Conditions: Column: 1.6 Ã 8.8 cm with ToyoPearl SP 650M. Mobile Phase: 150mM Citrate, Solute Pulse: 500 mL of 10 mg/mL globular protein.
Moments can be calculated by numerical integration, but care must be taken with the tails of the curves, especially for the second (and even more, the third). Here small errors are magnified at large time through multiplication by the square or cube of t . Extrapolations to infinite time are usually made from simple curve fits of the large-time data, and it often is sufficient to assume exponential decay with respect to t . One property of M 0 is that it is independent of system size and so is the same for the column proper and the whole experimental system (See Ref. 10, Ex. 23.6-3, for limitations on the use of moments. These are seldom important for our present purposes).
Table 1. ASTM Bases of N Determination
USING MOMENTS
To understand the benefits that the method of moments can offer, we base this part of our discussion on Figure 4, experimental data for a representative globular protein (molecular weight of about 150 kDa). The number of plates is calculated both by the method of moments (Equation 15), and by the widely used short-cut technique (at 50% of peak height from Table 1). The method of moments is seen to approach a straight-line asymptote on this figure, indicating that dispersion increases a bit faster than linearly with percolation velocity. This is in agreement with well-established theory as well as with common observation. To characterize the extent of dispersion, redefine plate number as per Equations (15) and (16). 11
The slope of a straight line from the origin to any point on this correlation (defined in Equation 17) can provide useful information for column scale-up. If we substitute Equation (17) and (18) into Equation (19), we are left with Equation (20).
The truest measure of column effectiveness is the number of plates, and Schneider found this to be proportional to solvent residence time, and inversely proportional to the slope g. It suggests, correctly, that solvent residence time is a safe scale-up criterion if velocity is no higher in the larger column, and the penalty for higher velocity can be predicted from the van Deemter plot of HETP as a function of u. Maintaining both solvent residence time and percolation velocity is almost always a reliable scale-up criterion — even for affinity columns with their notoriously slow kinetics. Moreover the effect of increasing velocity at constant solvent residence time is modest under most commonly observed conditions (high Pé).
On the other hand, the short-cut method falsely suggests that higher velocities yield a very large dividend on scale-up. They do not, and high velocities require expensive pumps and massive column walls. This is especially true for compressible particles.
CHARACTERIZING COLUMN FLOW DISTRIBUTION
We consider the problem of characterizing column packing. Poor flow distribution, resulting from either the column flow distributors or packing heterogeneity, can cause substantial deviations from the optimal performance of a chromatographic column. In a typical chromatographic process, flow in the distributors and the packed bed operates at very low Reynolds numbers, or in a laminar mode called creeping flow. Creeping flow through porous media is described by the pseudo-continuum Blake-Kozeny equation, presented here as Equation (21). 10
Reversing the pressure drop, which corresponds to a reversal of the direction of flow, corresponds only to a change in the sign but not the magnitude of the velocity. For this reason, creeping flow is said to be reversible. It should be noted that this equation and the derived reversibility is valid only for a distance scale that is large in comparison to d p . Characterizing heterogeneity can therefore be made with surprising ease via order-of-magnitude analysis because it usually occurs on a size scale, which is large compare to packing particle diameters.
The intrinsic broadening of a solute pulse, as predicted from linear chromatographic models, operates on a distance-scale comparable to a particle diameter, and it is irreversible. Thus, we may conclude that plate height is the sum of the dispersive contributions. All these dissipative processes occur independently of the flow direction.
These differences provide a very powerful tool for determining the quality of the flow distribution. Visualize a perfectly packed column with perfect headers, operated under normal conditions until a tracer pulse is halfway to the normal outlet, and then the flow is reversed — the effluent will now appear at the inlet. The pulse will have the same form as that leaving the full column under normal operation — small-scale contributions to band broadening will be the same as for normal operation. However, as per Equation (21), even if there is large-scale maldistribution of flow, the reversed-flow effluent will be that of a perfectly packed column with perfect headers.
A comparison of a pulse injected under normal conditions and under flow reversal at one-half of the retention time is shown in Figure 5. Flow reversal at one-half of the retention time mimics the effluent distribution of a perfectly packed column with perfect headers. The conventional, or forward-flow, effluent curve exhibits significant tailing for the system and column, which is not seen under reverse-flow conditions. This chromatographic column is only operating at 70% of its potential efficiency as determined by a comparison on the number of plates between the forward-flow and the reverse-flow case.
Figure 5. Comparison of Forward and Reverse-flow of an Acetone Pulse The conventional forward flow and reverse flow effluent curves have a plate count of 548 and 788. respectively.
A further extension of this reverse-flow technique has been developed that allows for the decoupling of the effects of non-uniform packing from poor distributor flow, with the latter solely dictated by the column design. 12 This extension provides a non-destructive test to characterize flow distribution.
FRONTAL ANALYSIS
In the majority of columns, performance is evaluated only once after the column is packed. Columns are often used multiple times, and it is essential to maintain packed- bed quality and efficiency throughout the column lifetime. Performing tracer analysis to measure the number of plates before every run can be time consuming and impractical for pilot- or commercial-scale columns. Frontal analysis, where a step change is applied to the packed bed rather than a pulse, is frequently used to estimate column performance without the need for extra lines or extra processing time.
It is easy to apply the established plate theory for linear chromatography to the response from a pulse. For linear systems, the response to a pulse input is equal to the derivative of the response to a step input. 13 Thus numerical differentiation of the monitored output will provide the same information as a response to a non-interacting tracer pulse. This is shown in Figure 6, where the response to a pulse and the differentiated response to a step are overlaid. Both chromatograms show similar responses, particularly in the peak front. The differentiated step response shows a slight deviation at the tail, which would result in a slightly higher HETP.
Figure 6. Comparison of Column Performance as Determined from the Response to a Salt Pulse and a Salt Front. Column 10 Ã 29 cm PhenylSepharose FF(High Sub). Salt Pulse Conditions: Mobile Phase: 400 mM Citrate, Solute Pulse, 50 mL of 150mM Citrate. Salt Front Conditions: Mobile Phase: 1: 400 mM Citrate, Mobile Phase II, 150 mM Citrate. Chromatograms rescaled to overlay.
The two distributions contain the same information, but the curve for the differentiated step is more easily obtained. The situation for non-linear distributions is more complicated, but again the differential curve is generally more useful.
Nomenclature
In summary, the method of moments when applied to the reverse-flow technique allows for a non-intrusive and accurate method to determine the quality of flow distribution. This is particularly useful for preparatory or commercial columns, where column packing is a tedious and expensive task. The use of statistical moments to evaluate column performance can provide a great deal of information on column performance with little effort.
Anurag Rathore is a principal scientist at Amgen Inc., 30W-2-A, One Amgen Center Drive, Thousand Oaks, CA 91320, 805.447.4491, fax 805.499.5008, [email protected] .
John Moscariello is a scientist at Amgen Inc., Seattle, WA.
Edwin Lightfoot is a professor at Department of Chemical and Biological Engineering, University of Wisconsin, Madison.
REFERENCES:
1. Martin AJP, Synge ELM. A new form of chromatogram employing two liquid phases. I. A theory of chrom-atography. 2 Application of the micro-determination of the higher monoamino-acids in proteins. Biochem. J. 1941; 35:1358.
2. Giddings JC, Eyring H. A molecular dynamic theory of chromatography. J. Phys. Chem . 1955; 59:416.
3. Giddings JC. The random downstream migration of molecules in chromatography. J. Chem. Ed . 1958; 35:588.
4. ASTM, March 1992, E 682.
5. Coffman JL. Protein diffusion in chromatographic media. doctoral thesis, U. Wisconsin. 1994.
6. Horváth CS, Melander WR, in Heftmann E (ed.). Theory of chromatography. Chromatography: fundamentals and applications in chromatographic and electropho-retic methods. Part A: Fund-amentals and techniques. New York, Elsevier Scientific; 1983:27.
7. Giddings JC. Generation of variance, theoretical plates, resolution, and peak capacity in electrophoresis and sedimen-tation. Sep. Sci. 1969; 4:476.
8. Giddings JC. Dynamics of chromatography. New York, Marcel Dekker 1965.
9. Lightfoot EN, Coffman JL, Lode F, Yuan QS, Perkins TW, Root TW. Refining the scale-up of chromatographic separations. J. Chrom A . 1997; 760: 139.
10. Bird, RB, Stewart WE, Lightfoot EN. Transport Phenomena. New York, Wiley; 2002.
11. Scheinder P, Smith JM. Adsorption rate constant form chroma-tography. AICHE J. 1968; 14:762.
12. Moscariello JS, Purdom G, Coffman JL, Root TW, Lightfoot EN. Characterizing the performance of industrial-scale columns. J. Chrom. A. 2001; 908:131.
13. Hill CG. An introduction to chemical engineering kinetics and reactor design. New York, Wiley; 1977.
Related Content:
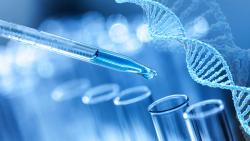
Set-up of 1D-column experiment.

Contexts in source publication
Similar publications.

- Hannia Hernández-Aguilar
- Carlos M. García-Lara

- APPL SURF SCI

- Recruit researchers
- Join for free
- Login Email Tip: Most researchers use their institutional email address as their ResearchGate login Password Forgot password? Keep me logged in Log in or Continue with Google Welcome back! Please log in. Email · Hint Tip: Most researchers use their institutional email address as their ResearchGate login Password Forgot password? Keep me logged in Log in or Continue with Google No account? Sign up

An official website of the United States government
The .gov means it’s official. Federal government websites often end in .gov or .mil. Before sharing sensitive information, make sure you’re on a federal government site.
The site is secure. The https:// ensures that you are connecting to the official website and that any information you provide is encrypted and transmitted securely.
- Publications
- Account settings
- My Bibliography
- Collections
- Citation manager
Save citation to file
Email citation, add to collections.
- Create a new collection
- Add to an existing collection
Add to My Bibliography
Your saved search, create a file for external citation management software, your rss feed.
- Search in PubMed
- Search in NLM Catalog
- Add to Search
Soil Column Experimental Study on the Effect of Soil Structure Disturbance on Water Chemistry
Affiliations.
- 1 Key Laboratory of Shale Gas and Geoengineering, Institute of Geology and Geophysics, Chinese Academy of Sciences, Beijing 100029, China.
- 2 College of Earth and Planetary Sciences, University of Chinese Academy of Sciences, Beijing 100049, China.
- PMID: 36497748
- PMCID: PMC9738350
- DOI: 10.3390/ijerph192315673
The changes in soil/rock structure caused by engineering disturbance or earthquakes could affect water chemistry by increasing the reaction surface, enhancing the oxidation condition, or exposing soluble rocks. However, the details of the mechanisms of the disturbance of soil/rock are little known. Based on the soil column experiment, this study analyzed the concentrations of sulfate (SO 4 ), sulfur, and oxygen isotopic composition of SO 4 (δ 34 S-SO 4 and δ 18 O-SO 4 ) in effluent water. The water-rock interaction mechanisms in the disturbed soil and the contribution of this interaction to the SO 4 in groundwater were studied. The results suggest that the concentration of SO 4 in the first effluent water sample can reach up to 97 mg/L, much higher than that in natural groundwater (6.8 mg/L). The isotopic composition of SO 4 further suggested that SO 4 in the first effluent water sample was mainly derived from the dissolution of SO 4 -containing evaporites. The proportion was estimated to be 93%. SO 4 -containing evaporites accounted for 23% of the SO 4 content in all effluent water samples during the experiment. The disturbance of soil structure led to the exposure and dissolution of SO 4 -containing evaporites, which were initially insoluble under natural conditions. This study is essential to the clarification of the water-rock interaction mechanisms following the changes in soil/rock structures.
Keywords: loess; soil column experiment; sulfate; sulfur and oxygen isotopes; water chemistry; water–rock interaction.
PubMed Disclaimer
Conflict of interest statement
The authors declare no conflict of interest.
Schematic diagram of soil column…
Schematic diagram of soil column structure.
Change in concentrations of SO…
Change in concentrations of SO 4 and δ 34 S-SO 4 in the…
Sulfur and oxygen isotope distribution…
Sulfur and oxygen isotope distribution in the effluent water (the ranges of the…
Conceptual diagram of the occurrence…
Conceptual diagram of the occurrence of SO 4 -containing evaporites and rock-water interaction…
Similar articles
- Tracing sulfate origin and transformation in an area with multiple sources of pollution in northern China by using environmental isotopes and Bayesian isotope mixing model. Zhang Q, Wang H, Lu C. Zhang Q, et al. Environ Pollut. 2020 Oct;265(Pt B):115105. doi: 10.1016/j.envpol.2020.115105. Epub 2020 Jun 26. Environ Pollut. 2020. PMID: 32806467
- δ34S and δ18O of dissolved sulfate as biotic tracer of biogeochemical influences on arsenic mobilization in groundwater in the Hetao Plain, Inner Mongolia, China. Li MD, Wang YX, Li P, Deng YM, Xie XJ. Li MD, et al. Ecotoxicology. 2014 Dec;23(10):1958-68. doi: 10.1007/s10646-014-1310-y. Epub 2014 Aug 23. Ecotoxicology. 2014. PMID: 25149868
- Source and evolution of sulfate in the multi-layer groundwater system in an abandoned mine-Insight from stable isotopes and Bayesian isotope mixing model. Mao H, Wang C, Qu S, Liao F, Wang G, Shi Z. Mao H, et al. Sci Total Environ. 2023 Feb 10;859(Pt 2):160368. doi: 10.1016/j.scitotenv.2022.160368. Epub 2022 Nov 19. Sci Total Environ. 2023. PMID: 36414065
- Effects of mining activities on evolution of water quality of karst waters in Midwestern Guizhou, China: evidences from hydrochemistry and isotopic composition. Li X, Wu P, Han Z, Zha X, Ye H, Qin Y. Li X, et al. Environ Sci Pollut Res Int. 2018 Jan;25(2):1220-1230. doi: 10.1007/s11356-017-0488-y. Epub 2017 Oct 29. Environ Sci Pollut Res Int. 2018. PMID: 29082473
- Research Advances in Identifying Sulfate Contamination Sources of Water Environment by Using Stable Isotopes. Wang H, Zhang Q. Wang H, et al. Int J Environ Res Public Health. 2019 May 30;16(11):1914. doi: 10.3390/ijerph16111914. Int J Environ Res Public Health. 2019. PMID: 31151187 Free PMC article. Review.
- Li Z.Q., Qi Z.Y., Qi S.W., Zhang L.X., Hou X.K. Microstructural changes and micro–macro-relationships of an intact, compacted and remolded loess for land-creation project from the Loess Plateau. Environ. Earth Sci. 2021;80:593. doi: 10.1007/s12665-021-09872-4. - DOI
- Zhang L.X., Qi S.W., Ma L.N., Guo S.F., Li Z.Q., Li G.L., Yang J.J., Zou Y., Li T.L., Hou X.K. Three-dimensional pore characterization of intact loess and compacted loess with micron scale computed tomography and mercury intrusion porosimetry. Sci. Rep. 2020;10:8511. doi: 10.1038/s41598-020-65302-8. - DOI - PMC - PubMed
- Bouma J. Influence of soil macroporosity on environmental quality. Adv. Agron. 1991;46:1–37.
- Kumar P., Mahajan A.K., Kumar A. Groundwater geochemical facie: Implications of rock-water interaction at the Chamba city (H.P.), northwest Himalaya, India. Environ. Sci. Pollut. Res. 2020;27:9012–9026. doi: 10.1007/s11356-019-07078-7. - DOI - PubMed
- Speight J.G. Water Chemistry. In: Speight J.G., editor. Natural Water Remediation: Chemistry and Technology. Butterworth-Heinemann; Boston, MA, USA: 2020. pp. 91–129. - DOI
Publication types
- Search in MeSH
Related information
- PubChem Compound (MeSH Keyword)
Grants and funding
Linkout - more resources, full text sources.
- Europe PubMed Central
- PubMed Central
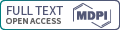
- Citation Manager
NCBI Literature Resources
MeSH PMC Bookshelf Disclaimer
The PubMed wordmark and PubMed logo are registered trademarks of the U.S. Department of Health and Human Services (HHS). Unauthorized use of these marks is strictly prohibited.
Tracking the Transport of Silver Nanoparticles in Soil: a Saturated Column Experiment
- Open access
- Published: 01 October 2018
- Volume 229 , article number 334 , ( 2018 )
Cite this article
You have full access to this open access article
- Karrar N. M. Mahdi 1 , 2 ,
- Ruud Peters 3 ,
- Martine van der Ploeg 1 ,
- Coen Ritsema 1 &
- Violette Geissen 1
3504 Accesses
28 Citations
2 Altmetric
Explore all metrics
Silver nanoparticles (AgNPs) can enter the environment when released from products containing them. As AgNPs enter soil, they are often retained in the soil profile and/or leached to the groundwater. This research assessed the transport of AgNPs in their “particle form” through the soil profile using a series of columns. Three soil types were put into soil columns: LSH (loam with high organic matter (OM)), LSL (loam with low OM), and Sand (no OM). The results showed that AgNP transport and retention in soil as well as particle size changes are affected by soil organic matter (OM) and the cation exchange capacity (CEC) of soil. OM affected the transport and retention of AgNPs. This was evident in the LSH columns where the OM concentration was the highest and the AgNP content the lowest in the soil layers and in the effluent water. The highest transported AgNP content was detected in the Sand columns where OM was the lowest. CEC had an impact on the particle size of the AgNPs that were retained in the soil layers. This was clear in columns packed with high CEC-containing soils (LSL and LSH) where AgNP particle size decreased more substantially than in the columns packed with sand. However, the decrease in AgNP sizes in the effluent water was less than the decrease in particle size of AgNPs transported through but retained in the soil. This means that the AgNPs that reached the effluent were transported directly from the first layer through the soil macropores. This work highlights the ability to track AgNPs at low concentrations (50 μg kg −1 ) and monitor the changes in particle size potential as the particles leach through soil all of which increases our knowledge about AgNP transport mechanisms in porous media.
Similar content being viewed by others
Retention of silver nanoparticles and silver ion to natural soils: effects of soil physicochemical properties.
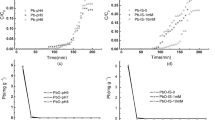
Retention of nano PbO in saturated columns and its dissolution kinetics in soils
Silver nanoparticles in soil: input, transformation, and toxicity, explore related subjects.
- Environmental Chemistry
Avoid common mistakes on your manuscript.
1 Introduction
Silver nanoparticles (AgNPs) are a metallic nanomaterial consisting of spherically shaped particles ranging in size from 1 to 100 nm (Helmlinger et al. 2016 ). AgNP production is growing fast and thus, so is the production of products and the development of applications that incorporate AgNPs (López-Serrano et al. 2014 ). Previous studies have researched the potential toxicity of AgNPs to humans and the environment (EC 2017 ). The increase in the production of AgNPs and the number of applications that use these AgNPs has raised concerns about the potential release of these materials into the environment during production, use, and inevitable disposal (Gottschalk and Nowack 2011 ; Yu et al. 2013 ).
AgNPs enter the soil environment through several different pathways including natural processes, like biological and chemical reduction of ionic silver, as well as anthropogenic activities, which seem to be the most significant sources (Yin et al. 2015b ). AgNPs can reach the soil through plant strengthening agents (Lorenz et al. 2011 ; Prasad et al. 2014 ; Thuesombat et al. 2014 ), slag from incinerators (Holder et al. 2013 ), and contaminated sludge from sewage treatment plants (Lombi et al. 2013 ). In Germany, around 30% of the annual sewage sludge solids produced (2 million tons per year) from municipal wastewater treatment plants are used for farmland application (Schlich et al. 2013a ). Similar amounts are used in Spain, Portugal, France, and the UK (Wiechmann et al. 2012 ). Furthermore, previous studies have shown that there is a large variation in the concentration and the particle size of the AgNPs that are used in consumer products which means that the different particle sizes and concentrations of AgNPs released into soil and water have different toxic effects. For example, the particle size of AgNPs used in a nasal spray is 28.9 nm at a concentration of 10 mg L −1 while an antifungal spray has AgNP with a particle size of 15.4 nm and a concentration of 30.2 mg L −1 (Guo et al. 2015 ). Once AgNPs are introduced to the soil environment, the AgNPs can be transported to deeper layers in the soil and to the groundwater (Sagee et al. 2012 ; Cornelis et al. 2013 ; Liang et al. 2013a ) as well as transported with surface water runoff (Kaegi et al. 2010 ; Tian 2010 ; Mahdi et al. 2017 ).
AgNPs that are released to the soil environment may encounter changes in physical-chemical properties as they interact with the soil (Yin et al. 2015a ). Therefore, soil characteristics can affect AgNP behavior in soil. For example, the presence of organic matter (OM) is believed to prevent AgNPs from dissolution as they coat the AgNP particles which inhibits the release of Ag + (Klitzke et al. 2014 ) and can make the AgNPs less mobile in the soil (Coutris et al. 2012 ). Other studies have shown that the retention of nanomaterials generally increases, and the bioavailability decreases in soils with a finer grain size distribution (Shoults-Wilson et al. 2011a ; Cornelis et al. 2012 ). The cation exchange capacity (CEC), ionic strength (IS), pH, and soil particle size distribution also have an impact on the fate and transport pattern of AgNPs in soil (Lowry et al. 2010 ; Liang et al. 2013a ). Therefore, the complexity of the soil system makes it difficult to understand the transport and distribution of AgNPs in soils (Pan and Xing 2012 ).
In relation to toxicity, many studies have shown that smaller particle sizes of AgNPs result in higher toxicity effects (Pal et al. 2007 ; Kim et al. 2012 ; Silva et al. 2014 ). Furthermore, the AgNPs that are applied in a pristine form or in a transformed form (due to interactions with other soil chemicals) have both proven to be toxic to soil species (Zhang et al. 2012 ; Schlich et al. 2013b ). Schlich et al. ( 2013b ) also showed that AgNPs applied to soil at low concentrations could be toxic to soil microorganisms.
Detection of AgNPs in soil is complicated and requires very sensitive instruments and careful methods (Yu et al. 2015 ). Generally, Ag concentration in soil has been determined via the acidic digestion of the soil samples (EPA 1996 ). However, since AgNPs dissolve in this procedure, any information about the presence of AgNPs in the soil sample is lost and nothing can be concluded about the stability, transportation, and transformation of AgNPs in soil.
This study focused on tracking the transport and the changes in particle size of AgNPs, as they moved through 16-cm-long columns filled with different soils and in the effluent eventually leached from these columns. We used the saturated soil column method modified to mimic the real-world application of sludge-containing AgNPs and possible leaching scenarios. AgNP transport in soil increases with the increased application of rainwater (RW); therefore, RW was applied in periods of 24, 48, and 72 h in an attempt to observe the transport pattern of AgNPs in the soil and effluent water (EW) that was sampled after each RW application. The detection and characterization of AgNPs in soil was achieved using a combination of an aqueous extraction method and the single particle inductively coupled plasma mass spectrometry method (spICP-MS). This combination had the capability of detecting very low AgNP concentrations in the soil (Peters et al. 2015 ; Mahdi et al. 2016 ). The results obtained from this work contribute to filling in the knowledge gap regarding AgNP transport and leaching through soil and increases the awareness of AgNP distribution patterns in soil. These findings can be used to improve understanding and aid in the management of products and waste containing AgNPs.
2 Materials and Methods
2.1 materials, 2.1.1 soils and rainwater.
Three types of soil were used in this study and were provided by Unifarm (Wageningen, the Netherlands). The soils used had the following characteristics: loam with high organic matter (LSH), loam with low organic matter (LSL), and sand with no OM content (Sand). The initial characteristics of the soil are given in Table 1 .
The simulated rain was applied via a sprinkler on the top of the soil column. The rainwater setup is described in detail in the experimental design section ( 2.2.1 ). The pH and IS of the water were measured and were 7.63 and 3.8 mM respectively.
2.1.2 Chemicals
The AgNPs used consisted of an aqueous suspension of citrate-stabilized spherical AgNPs from NanoComposix (Prague, Czech Republic). The particle diameter was 60 nm and the particle mass concentration in the aqueous suspension was 1000 mg L −1 .
Potassium Bromide
A potassium bromide (KBr) solution with a concentration of 0.0167 M was purchased from Fisher Scientific (Landsmeer, the Netherlands). KBr was used as an inert tracer in the experiments.
2.2 Experimental Method
This column experiment was conducted in the soil physics laboratory of Wageningen University in the Netherlands. There were three replicates for each soil type and time period (24, 48, and 72 h), resulting in a total of 27 columns. Analyses of samples collected from the soil columns and leachate (effluent water) was performed at RIKILT Wageningen University and Research in the Netherlands. Figure 1 shows a schematic overview of the experimental design and a flow chart of the steps taken during the experiment.
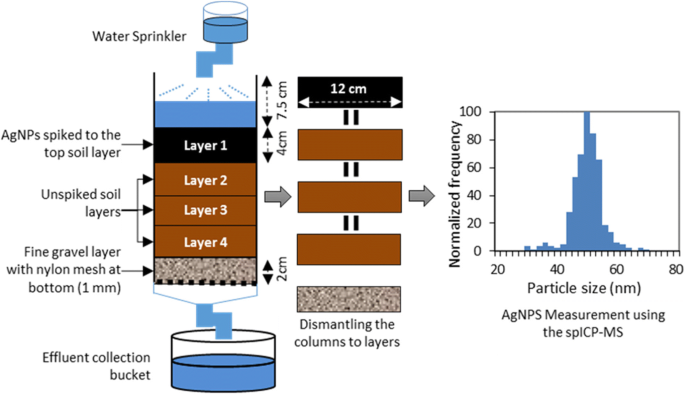
The experimental design of the column experiments
2.2.1 Soil Column Setup
Soil column preparation.
The experiment was conducted using a series of polyethylene (PE) hydraulic soil columns with a diameter of 12 cm and depth of 25.5 cm. The bottom of each column was covered with a thin nylon mesh with apertures (< 1 mm). Prior to the experiment, all soil types used (LSH, LSH, and Sand) were air dried at room temperature (for 7 days) and sieved using sieve #5 (apertures size was 4 mm) to keep the soil properties close to the initial soil.
Before filling the columns with soil, a 2-cm layer of fine gravel (2–4 mm) was added to the bottom (to work as a filter for soil particles from the column). Then, each column was filled up to 12 cm of soil representing three layers (each layer ± 4 cm). While adding the soil, the column wall was gently tapped to minimize air entrapment and to achieve homogenous soil packing. With the three lower soil layers in place, the columns were saturated with three pulses of RW. Finally (after 24 h), a top layer spiked with AgNPs was added as layer 1, ensuring that it had the same moisture content as the other soil layers in the soil column.
Rainwater Pulses
RW was applied to the top of the columns in pulses. Each pulse equaled 1 pore volume (PV) of the soil in the particular column. PV was determined from the total porosity of the soil samples which was considered to be equal to the volumetric soil water content at saturation. Therefore, to bring the soil in a column to the saturation level, a volume of water equal to the difference between dry moisture and the saturation level was necessary. The calculated porosities of the LSH and LSL soil columns were not significantly different. Thus, a fixed 42.2%, PV value equal to the average pore volume of these soils was used. To avoid problems related to inconsistent calculations of the AgNP concentration collected from different columns, this PV value was also applied to the Sand columns.
RW was applied using an inverted volumetric flask fixed above the column and connected to a sprinkler. The RW application rate was such that a constant amount of water was maintained on the top layer. Following addition of the AgNP-spiked soil to the columns, 1 RW pulse was applied per day during the experiment.
Bromide Tracer Breakthrough Check
To confirm that the column setup was functioning well, bromide (Br) was used as a soluble conservative tracer to test breakthrough volumes. Breakthrough data is an indicator of the hydrodynamic properties of the soil column.
Three columns for each soil type were tested before the experiment. The columns were prepared in the same manner as described above with the exception that the top layer (layer 1) was not spiked with AgNPs. Each column was first saturated with 3 RW pulses. Then, three pulses containing 20 mg L −1 Br were applied followed by another three pulses of RW to remove all Br from the column. Bromide concentrations were detected using an inductively coupled plasma mass spectrometer (ICP-MS) in standard mode (Creed et al. 1996 ). A schematic flow chart depicting the steps during the experiment is shown in Fig. 2 .
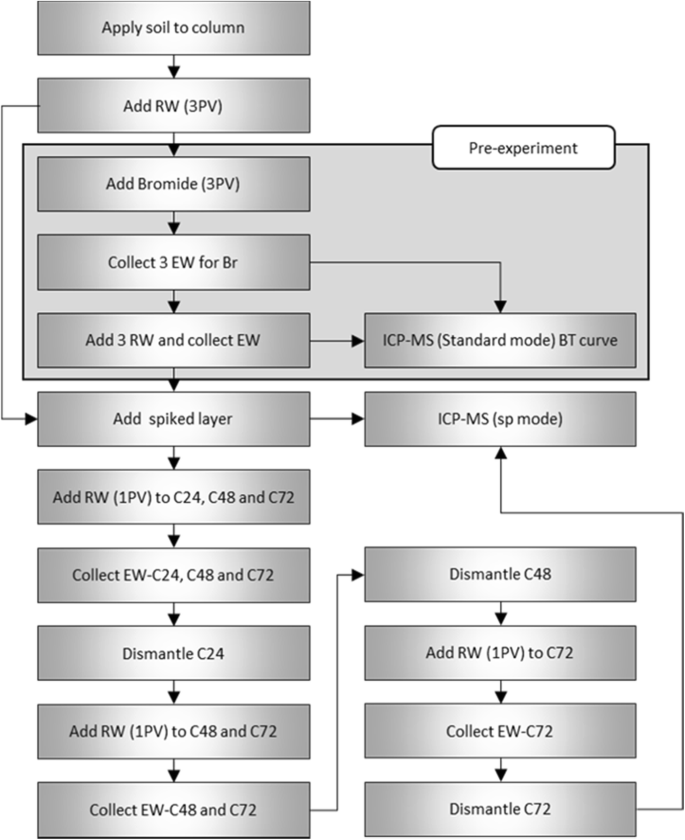
Schematic flow chart of the steps in the experiment. RW is rainwater; PV is pore volume; EW is effluent water; C is column series; the numbers 24, 48, and 72 depict the residence time in the column. The flow chart represents one replicate for one soil type
2.2.2 AgNP Application
The AgNP-spiked soil that was added to the columns (as layer 1) was prepared by diluting the AgNP stock solution to get a net concentration of 50 μg kg −1 with an average particle size equal to ± 60 nm. Milli-Q water (MQW) produced by an Ultrapure Water system from Millipore (Amsterdam, the Netherlands) was used for diluting AgNP spikes, collecting AgNP samples and preparing all other additional chemicals. The AgNP-spiked solutions (in volumes equal to 1 PV) were mixed with the dry-sieved soil (± 400 g) for soil layer 1 which was then saturated to match the saturation of the other soil layers in the column. As previously noted, the soil spiked with AgNPs was added as the top soil layer (layer 1) for each column. After the addition of this layer, the columns were left in the dark to equilibrate for 24 h.
2.2.3 Sample Collection and Characterization
Sample collection.
During the experiment, the collection of the effluent water (EW) containing leached AgNPs was started after the addition of each RW pulse to a column and continued for 24 h. For column series 1 (C24), only 1 volume of EW was collected. For column series 2 (C48), 2 EW volumes were collected and for column series 3 (C72), 3 were collected. EW samples were collected using dark-glass flasks and stored at room temperature.
Soil samples were collected from the dismantled columns. Column series 1 (C24) was dismantled after 1 RW pulse (24 h), column series 2 (C48) was dismantled after 2 RW pulses (48 h), and column series 3 (C72) was dismantled after applying 3 RW pulses (72 h).
Columns were carefully dismantled and sliced into the four layers 1 to 4, each 4 cm thick. Each individual soil slice was homogenized and 3 sub-samples < 10 g were collected for the determination of AgNPs. This resulted in 36 samples per soil type. For soil property determination, 3 samples per soil layer (weight of the sample < 50 g) were taken, totaling 12 samples per soil type.
Other samples such as RW, initial soil, and AgNP concentration control samples were measured simultaneously with the samples (soil and EW) containing AgNPs from the columns to get a clear image for AgNP concentration and particle size calculations. In total, more than 100 soil and liquid (EW or RW) samples were collected for each soil type for AgNP detection and soil characterization.
Soil Property Characterization
Soil characteristics were determined both before the experiments (Table 1 ) and after dismantling the columns. Soil texture for the three soil types was analyzed using a laser diffraction technique at the University of Leuven (Leuven, Belgium). Soil pH was measured using a portable probe (WTW pH 340) obtained from WTW company (Weilheim, Germany). CEC (Ca + 2 and K + ) were analyzed by NIRs (near infrared spectroscopy) based on 0.0166 M cobalthexamine trichloride (ISO 1994 ) in the Coimbra College of Agriculture (Coimbra, Portugal). IS was derived directly from the electrical conductivity (EC) using the formula of the linear approach method (aqion 2016 ). EC itself was measured using a portable probe WTW pH 340i (WTW, Weilheim, Germany). Organic matter (OM) content was measured according to the standard ASTM method (ASTM 2000 ).
AgNP Quantification
AgNP concentration and particle size characteristics in soil samples and EW were quantified. AgNPs in soil samples were first extracted using the aqueous extraction method as described by Mahdi et al. ( 2016 ). In EW, AgNPs were extracted by settling and dilution to remove the leached-out soil particles and OM.
After extraction, AgNP samples were measured for concentration and particle size using the single particle inductively coupled plasma mass spectrometry method (spICP-MS). The inductively coupled plasma mass spectrometer (ICP-MS) used to perform the spICP-MS was a Thermo Scientific Xseries-2 from (MA, USA). The instrument and technical settings for spICP-MS have been described previously by Peters et al. ( 2015 ). All measurements were performed at RIKILT Wageningen University & Research (Wageningen, the Netherlands).
2.2.4 AgNP Mass Balance Calculations
The mass balance for the AgNPs transported in the soil columns was calculated based on the initial amount applied to layer 1 of each column and the final AgNP content detected in the soil layers (1, 2, 3, and 4), and the effluent water after each rainwater application. The total mass balance was calculated for AgNP content after the third rain application for all soil layers. EW samples were expressed in micrograms and compared with initial AgNP contents applied.
3.1 Soil Layer Characterization
After the experiment, each soil layer was used to assess the selected soil properties. The results shown in Table 2 indicate that the soil properties in all soil layers were more or less identical. So, no significant differences were found between the soil layers for pH, OM, CEC, and IS.
3.2 Performance of Soil Columns
The bromide tracer experiment revealed different breakthrough curves (see Fig. 3 ). Columns packed with sand showed the fastest and highest breakthrough and columns packed with LSL the slowest and lowest. Breakthrough curves of LSH showed an intermediate pattern.
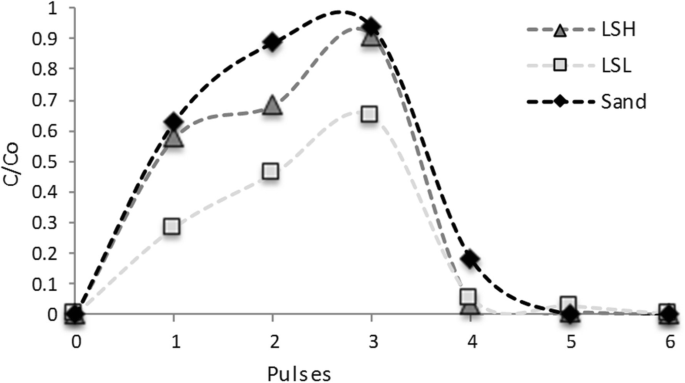
Breakthrough curves for bromide in the soil columns. LSH, column packed with loam soil with high organic matter; LSL, column packed with loam soil with low organic matter; Sand, column packed with sand. C/Co is ratio of the detected concentration of Br to the initially applied concentration. The first three pulses contained Br (20 mg L −1 ) and the others (4, 5, and 6) only rain water
3.3 AgNP Transport in the Soil Columns
3.3.1 agnp concentration in the soil layers.
The levels of AgNPs detected in the soil layers are shown in Fig. 4 . In the columns packed with LSH, there was very limited AgNP transport after 24 h as less than 1 μg AgNPs was detected in each of the soil layers 2 and 3 and almost none in layer 4 while 20 μg of the initially applied AgNPs remained in layer 1. After 48 h, there was very little change in the transport of AgNPs compared with the 24 h results. However, after 72 h, AgNP content in layers 2, 3, and 4 increased; the detected amount in layer 2 increased to 2 μg, in layer 3 to 0.7 μg, and in layer 4 to more than 1.5 μg while AgNP concentration in layer 1 was reduced to 15 μg.
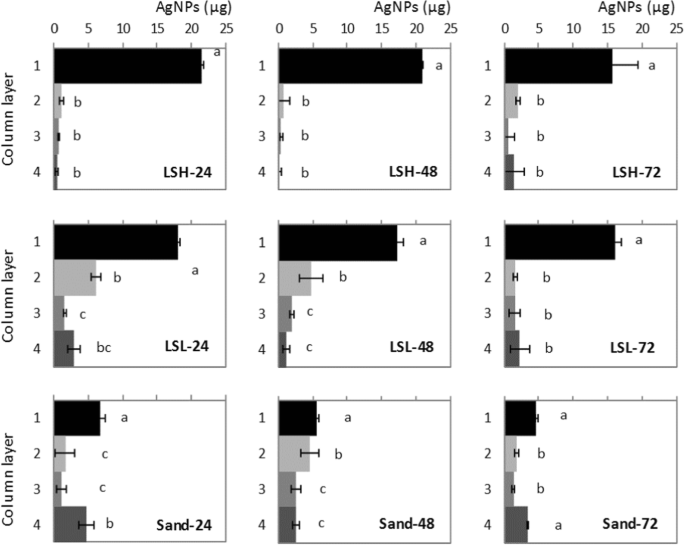
AgNP content in soil columns after the conclusion of the experiment. LSH, columns packed with loam soil with high organic matter; LSL, columns packed with loam soil with low organic matter; and Sand, columns packed with sandy soil; the numbers (24, 48, and 72) refer to the residence time of the AgNPs in the soil columns, and the time when they were dismantled to layers. Different lowercase letters indicate the significant differences ( p < 0.05) in AgNP content between soil layers for the same soil column
In the columns packed with LSL, the highest AgNP content was also found in layer 1. However, AgNPs detected in the soil layers 2, 3, and 4 were substantially higher than in the columns packed with LSH for all three sampling times (24, 48, and 72). The amount of AgNPs transported to the layers 2, 3, and 4 was the highest after 24 h and then decreased with time. Furthermore, AgNP content in layer 2 after 24 h was 6.2 μg, which was higher than the AgNP content in layers 3 and 4. However, after 72 h, AgNP content in layer 2 decreased to just 1.6 μg, which was lower than the AgNPs detected in layer 4.
In columns packed with sand, the AgNP transport pattern was different than that found in the LSH and LSL columns. In the Sand columns, layer 1 retained fewer AgNPs indicating that more AgNPs were transported to the deeper layers. After 24 h, AgNP content in layer 1 was 6.8 μg and in layer 4, it was 4.8 μg while in layers 2 and 3, the content was 1.6 μg and 1.1 μg, respectively. After 48 h, AgNP content in layers 2 and 3 increased to 4.5 μg in layer 2 and 2.6 μg in layer 3 while it dropped to 5.5 in layer 1 and 2.5 in layer 4. After 72 h, AgNP content in all layers decreased except in layer 4: in layer 1, it was < 5 μg; in layers 2 and 3, it was < 2 μg; while in layer 4, it was 3.4 μg. Furthermore, in several soil columns, we noticed a significant difference between AgNP content in layer 4 and layers 2 and/or 3. This is due to AgNP filtration problems from soil to gravel layers which was also the case in real scenarios (Kumahor et al. 2016 ).
3.3.2 AgNP Content in the Effluents
The results for the levels of AgNPs detected in effluent water (EW) are shown in Fig. 5 . In the EW from columns packed with LSH, less than 1 μg of AgNPs was detected for each of the three collection times (24, 48, and 72 h). The total AgNP content in EW from these columns (2.2 μg) was the lowest of the totals from all three soil types.
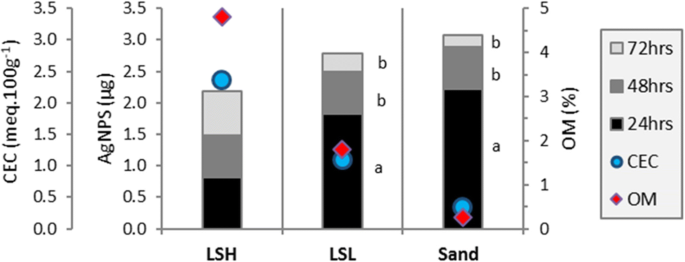
AgNP content detected in effluent water. LSH, columns packed with loam soil with high organic matter; LSL, columns packed with loam soil with low organic matter; Sand, columns packed with sandy soil; OM, organic matter (represented by the secondary Y -axis); and CEC, cation exchange capacity calculated for Ca +2 and K + . Different lowercase letters indicate the significant differences ( p < 0.05) in AgNP content in the three collection times of EW
For columns packed with LSL, AgNP content detected in the EW collected was highest after 24 h (± 1.8 μg) and then decreased significantly in the EW collected at 48 and 72 h, with only ± 0.3 μg detected at 72 h.
Similar to the results for the EW from the LSL columns, the AgNP content in EW from the Sand columns was highest after the 24-h collection and then decreased in the EW collected after 48 and 72 h. However, the amount detected after 24 h was greater than that detected in the LSL EW, making the total AgNP content collected from the sand EW the highest among all soil types.
Overall, the AgNP content in the EW increased from fine textured to coarse textured soil. As expected, there was an inverse relationship between OM and the AgNPs detected in the EW. A similar relationship existed for CEC.
3.3.3 Particle Size Tracking in the Columns and Effluent
The particle size of the AgNPs decreased continuously in the soil column layers as particles moved from the top to the bottom layers as shown in Fig. 6 . This was true for all soil types, although the amount of change differed between soils. In the LSH and LSL columns, AgNP particle size in layers 3 and 4 was reduced by 60–70% after 72 h of AgNP residence time in the columns. In Sand columns, the particle size reduction was 40–50% after 72 h.
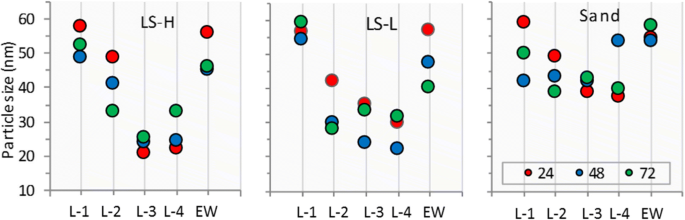
AgNP particle size in the columns. LSH, columns packed with loam soil with high organic matter; LSL, columns packed with loam soil with low organic matter column; sand, columns packed with sandy soil. (L-1 to L-4), soil layers; EW, column effluent; the numbers (24, 48, and 72) refer to the time of soil column dismantling and the time to collect the effluent
In the EW, AgNP particle sizes are generally larger than the ones found in soil layers 2 to 4, indicating the existence of flood paths facilitating rapid transport of particles from layer 1 to the effluent water. In the Sand columns, particle size decreased the least.
3.4 Total AgNPs Transported Through the Soil Columns
As shown in Fig. 7 , the total amounts of AgNPs transported from layer 1 to layers 2–4 and EW were 10.1%, 13.3% and 24.6% of the initial applied AgNPs in the LSH, LSL, and Sand columns respectively.
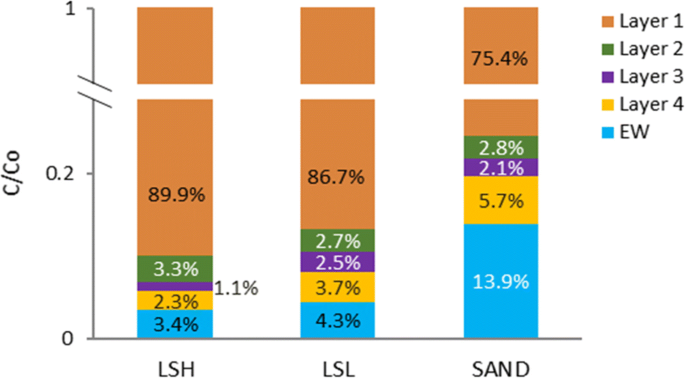
Total transported amount of AgNPs in the soil columns’ layers and in the effluents. C/Co is the ratio of the initial applied AgNP concentration to the detected concentration; LSH, columns contain loam soil with high organic matter; LSL, columns contain loam soil with low organic matter column; Sand, columns contain sandy soil. EW, the column effluents accumulated for the three times of collection
4 Discussion
4.1 agnp distribution in the soil layers.
AgNP transport patterns differ per soil and per layer as a result of the differences in OM, CEC, and soil texture.
In the columns packed with LSH, slow AgNP content transport was noticed during the first two collections (24 and 48 h). However, after 72 h, the amount of AgNPs detected had increased in all layers, especially in layers 2 and 4. This slow transport was attributed to the curtailment of AgNP transport due to the fine texture of LSH (compared to LSL and Sand) and to the related high organic matter and CEC content. Fine textured soil with high values of OM and CEC have the potential to increase AgNP adsorption on soil particles (Sagee et al. 2012 ; Coutris et al. 2012 ; Liang et al. 2013a ).
In the columns packed with LSL, faster AgNP transport (than in LSH columns) was noticed after applying the first RW pulse (samples collected after 24 h), causing relatively high concentrations in deeper soil layers and effluent water. This was due to the fact that LSL is less finely textured than LSH, which reduced the adsorption potential of AgNPs on soil particles, thus increasing AgNP mobility (Shoults-Wilson et al. 2011b ). The lower OM and CEC in LSL increased AgNP mobility as OM can inhibit AgNP mobility by coating the Ag particles and the lower CEC content can reduce cation bridging (Liang et al. 2013a ). After 48 and 72 h, only small differences in the AgNP content of layers 2 and 3 were noticed and the layers had almost the same AgNP content after 72 h.
In the columns packed with sand, the AgNP transport pattern was fast and unstable due to the coarse texture of the sand. Our findings confirm what has been reported by others (Sagee et al. 2012 ; Liang et al. 2013b ). In addition, there was no diminished AgNP transport due to low OM, CEC, or IS content.
Therefore, AgNP retention and/or mobility mechanisms inside the soil profile can be affected by the physical properties of soil such as particle size distribution and soil chemistry, like IS, OM, CEC, and pH. When soil particle sizes decrease, their surface area to volumes will increase which increase the attachment of AgNPs on these soil particles. Increasing attachment to soil particles means that AgNPs would experience restricted mobility and increasing effects from OM, CEC, IS, and pH. The existence of high OM would restrict the mobility of AgNPs due to increasing of AgNP attachments on OM. High CEC, as confirmed in this study, was found to have a stronger effect when AgNP particle size decreased. Regarding pH, we maintained a neutral pH in all soils used so that the mean pH would not play a role in the understanding of retention and mobility mechanisms of AgNPs inside soil (Yin et al. 2015a ). IS values, on the other hand, were the lowest in sand-packed columns. This enhanced AgNP mobility and transport due to the compression of the electric double layer (Liang et al. 2013a ).
4.2 AgNP Concentration in the Effluent Water
As with the distribution of AgNPs in the soil layers, AgNP content in EW was influenced by soil texture, OM, and CEC. As a result, coarsely textured media with a low OM content allowed the most rapid and distinct transport of AgNPs towards the bottom of the columns and resulted in high concentrations in effluent water.
4.3 Particle Size Distribution
AgNP particle sizes in the soil layers and in the EW were also monitored during the transport process. The particle size of AgNPs has been linked to the potential toxicity impact of AgNPs in many studies, with smaller particles expected to have a higher toxicity (Pal et al. 2007 ; Kim et al. 2012 ; Silva et al. 2014 ). In general, our results showed that AgNP particles became smaller as they moved through the soil over time and their new size depended on the soil type and soil depth. Figure 6 shows some differences in the AgNP sizes inside each individual column for layers 2, 3, and 4 but they were not significant. However, particle size differences for AgNPs retained in these layers and the AgNPs in layer 1 and in EW were significant.
In the columns packed with LSH and LSL soils, AgNP particle sizes decreased with time and depth due to chemical interactions with the soil particles. In the columns packed with sand, the AgNP particle sizes were more stable than in the LSH and LSL columns. While most of the particle size decreases occurred in the lower layers, some also occurred in layer 1. The AgNP concentration in layer 1 was far higher than in the other layers so the dissolution of AgNPs was less.
Yin et al. ( 2015a ) reported that AgNP particle size decrease was related to the AgNP properties (initial concentration, coating, particle size, etc.) and to the receiving soil medium (pH, IS, CEC, OM, soil type, and soil particle distribution). In the current study, only one type and one particle size of AgNPs was used so differences in AgNP particle sizes in this study are expected to have been the effect of soil characteristics. In general, AgNP particle size decrease occurs directly after AgNPs is mixed with water due to the formation of Ag 2 O which enhances the Ag + release (Sotiriou et al. 2012 ). Our results showed that Ag particle size in the soil layers was subjected to more dissolving than in the EW. The reason for this is that AgNP mobility in the columns packed with LSH and LSL soils was restricted due to the presence of OM and larger amounts of the fine-textured particles (clay and silt) compared to sand. This caused the AgNPs to be trapped in the pore voids of soil. This trapping also led to increased interaction between AgNPs and soil particle reactive compounds like OM and CEC.
In EW, AgNPs were transported “mostly” directly from layer 1 (where the AgNPs were applied) to the outlet which means that CEC and OM had less of an effect on particle size than what occurred in the soil layers. This was verified by following the time scans of Ag + signals in ICP-MS. In the time scan for layers 2, 3, and 4 of all columns, where the AgNP particle size decrease was higher than in layer 1, the higher signal of Ag + meant that there was a higher rate of dissolution. However, in layer 1 and the EW, the AgNP size decreased less and the Ag + signal was lower. This verifies that AgNPs in EW were mostly leached directly from layer 1.
5 Conclusion and Recommendations
AgNPs have been confirmed to be transported with water in soil columns which can lead to retention in deeper soil layers and leaching via effluent water despite the low (initial) concentrations applied. This may cause pollution.
The detection of AgNPs in soil layers and in the effluents confirms the potential risk of contamination resulting from AgNP application to soil.
AgNP retention in soil was affected by soil OM and AgNP particle size was affected by the CEC of soil.
The spICP-MS method allowed for the detection of AgNP particle size in soil layers and in the effluents, which revealed that AgNPs in the effluent were more stable in relation to size than in the soil layers which may impact water bodies.
Apart from the fact that AgNPs can be transported through soils, they may also be redistributed by runoff and erosion processes in undulated areas due to the strong adsorption of AgNPs to soil particles.
Further research on the transport of AgNPs in soil is needed focusing on lower concentrations of applied AgNPs, other AgNP particle sizes and other types of soil to assess the differences in transport dynamics and potential toxicity of the transported AgNPs on different soil species and plants.
aqion. Electrical Conductivity (EC). Retrieved December 10, 2016. http://www.aqion.de/site/130 2016 .
ASTM 2974. (2000). Standard test methods for moisture, ash, and organic matter of peat and other organic soils. American Society of Testing and Materials, 10 , D2974.
Google Scholar
Cornelis, G., DooletteMadeleine Thomas, C., McLaughlin, M. J., Kirby, J. K., Beak, D. G., & Chittleborough, D. (2012). Retention and dissolution of engineered silver nanoparticles in natural soils. Soil Science Society of America Journal, 76 , 891–902.
Article CAS Google Scholar
Cornelis, G., Pang, L., Doolette, C., Kirby, J. K., & McLaughlin, M. J. (2013). Transport of silver nanoparticles in saturated columns of natural soils. Science of the Total Environment, 463 , 120–130.
Article Google Scholar
Coutris, C., Joner, E. J., & Oughton, D. H. (2012). Aging and soil organic matter content affect the fate of silver nanoparticles in soil. Science of the Total Environment, 420 , 327–333.
Creed, J. T., Magnuson, M. L., Pfaff, J. D., & Brockhoff, C. (1996). Determination of bromate in drinking waters by ion chromatography with inductively coupled plasma mass spectrometric detection. Journal of Chromatography A, 753 , 261–267.
EC. (2017). Assessing the environmental safety of manufactured nanomaterials. In-depth report 14 produced for the European Commission, DG Environment by the science communication unit, UWE, Bristol. European Commission.
EPA. (1996). Method 3050B: acid digestion of sediments, sludges, and soils. SW-846 test methods for evaluating solid wastes.
Gottschalk, F., & Nowack, B. (2011). The release of engineered nanomaterials to the environment. Journal of Environmental Monitoring, 13 , 1145–1155.
Guo, H., Zhang, Z., Xing, B., Mukherjee, A., Musante, C., White, J. C., & He, L. (2015). Analysis of silver nanoparticles in antimicrobial products using surface-enhanced Raman spectroscopy (SERS). Environmental Science & Technology, 49 , 4317–4324.
Helmlinger, J., Sengstock, C., Groß-Heitfeld, C., Mayer, C., Schildhauer, T. A., Köller, M., et al. (2016). Silver nanoparticles with different size and shape: equal cytotoxicity, but different antibacterial effects. RSC Advances, 6 , 18490–18501.
Holder, A. L., Vejerano, E. P., Zhou, X., & Marr, L. C. (2013). Nanomaterial disposal by incineration. Environmental Science: Processes & Impacts, 15 , 1652–1664.
CAS Google Scholar
ISO. (1994). Soil quality—determination of effective cation exchange capacity and base saturation level using barium chloride solution (ISO 11260: 1994+ Cor 1: 1996).
Kaegi, R., Sinnet, B., Zuleeg, S., Hagendorfer, H., Mueller, E., Vonbank, R., et al. (2010). Release of silver nanoparticles from outdoor facades. Environmental pollution (Barking, Essex: 1987), 158 , 2900–2905.
Kim, T. H., Kim, M., Park, H. S., Shin, U. S., Gong, M. S., & Kim, H. W. (2012). Size-dependent cellular toxicity of silver nanoparticles. Journal of Biomedical Materials Research Part A, 100 , 1033–1043.
Klitzke, S., Metreveli, G., Peters, A., Schaumann, G. E., & Lang, F. (2014). The fate of silver nanoparticles in soil solution—sorption of solutes and aggregation. Science of the Total Environment, 535 , 54–60.
Kumahor, S. K., Hron, P., Metreveli, G., Schaumann, G. E., Klitzke, S., Lang, F., et al. (2016). Transport of soil-aged silver nanoparticles in unsaturated sand. Journal of Contaminant Hydrology, 195 , 31–39.
Liang, Y., Bradford, S. A., Simunek, J., Heggen, M., Vereecken, H., & Klumpp, E. (2013a). Retention and remobilization of stabilized silver nanoparticles in an undisturbed loamy sand soil. Environmental Science & Technology, 47 , 12229–12237.
Liang, Y., Bradford, S. A., Simunek, J., Vereecken, H., & Klumpp, E. (2013b). Sensitivity of the transport and retention of stabilized silver nanoparticles to physicochemical factors. Water Research, 47 , 2572–2582.
Lombi, E., Donner, E., Taheri, S., Tavakkoli, E., Jämting, Å. K., McClure, S., et al. (2013). Transformation of four silver/silver chloride nanoparticles during anaerobic treatment of wastewater and post-processing of sewage sludge. Environmental pollution (Barking, Essex: 1987), 176 , 193–197.
López-Serrano, A., Olivas, R. M., Landaluze, J. S., & Cámara, C. (2014). Nanoparticles: a global vision. Characterization, separation, and quantification methods. Potential environmental and health impact. Analytical Methods, 6 , 38–56.
Lorenz, C., Hagendorfer, H., von Goetz, N., Kaegi, R., Gehrig, R., Ulrich, A., et al. (2011). Nanosized aerosols from consumer sprays: experimental analysis and exposure modeling for four commercial products. Journal of Nanoparticle Research, 13 , 3377–3391.
Lowry, G. V., Hotze, E. M., Bernhardt, E. S., Dionysiou, D. D., Pedersen, J. A., Wiesner, M. R., et al. (2010). Environmental occurrences, behavior, fate, and ecological effects of nanomaterials: an introduction to the special series. Journal of Environmental Quality, 39 , 1867–1874.
Mahdi, K. N. M., Peters, R. J. B., Klumpp, E., Bohme, S., Van der Ploeg, M., Ritsema, C. J., et al. (2016). Silver nanoparticles in soil: aqueous extraction combined with single-particle ICP-MS for detection and characterization. Environmental Nanotechnology, Monitoring & Management, 7 , 24–33.
Mahdi, K. N. M., Commelin, M., Peters, R. J. B., Baartman, J. E. M., Ritsema, C. J., & Geissen, V. (2017). Transport of silver nanoparticles by runoff and erosion – a flume experiment. Science of the Total Environment, 601–602 , 1418–1426.
Pal, S., Tak, Y. K., & Song, J. M. (2007). Does the antibacterial activity of silver nanoparticles depend on the shape of the nanoparticle? A study of the gram-negative bacterium Escherichia coli. Applied and Environmental Microbiology, 73 , 1712–1720.
Pan, B., & Xing, B. (2012). Applications and implications of manufactured nanoparticles in soils: a review. European Journal of Soil Science, 63 , 437–456.
Peters, R., Herrera-Rivera, Z., Undas, A., van Marvin, H., Bouwmeester, H., der Lee, M., et al. (2015). Single particle ICP-MS combined with a data evaluation tool as a routine technique for the analysis of nanoparticles in complex matrices. Journal of Analytical Atomic Spectrometry, 30 , 1274–1285.
Prasad, R., Kumar, V., & Prasad, K. S. (2014). Nanotechnology in sustainable agriculture: present concerns and future aspects. African Journal of Biotechnology, 13 , 705–713.
Sagee, O., Dror, I., & Berkowitz, B. (2012). Transport of silver nanoparticles (AgNPs) in soil. Chemosphere, 88 , 670–675.
Schlich, K., Klawonn, T., Terytze, K., & Hund-Rinke, K. (2013a). Hazard assessment of a silver nanoparticle in soil applied via sewage sludge. Environmental Sciences Europe, 25 , 17.
Schlich, K., Klawonn, T., Terytze, K., & Hund-Rinke, K. (2013b). Effects of silver nanoparticles and silver nitrate in the earthworm reproduction test. Environmental Toxicology and Chemistry, 32 , 181–188.
Shoults-Wilson, W. A., Reinsch, B. C., Tsyusko, O. V., Bertsch, P. M., Lowry, G. V., & Unrine, J. M. (2011a). Effect of silver nanoparticle surface coating on bioaccumulation and reproductive toxicity in earthworms (Eisenia fetida). Nanotoxicology, 5 , 432–444.
Shoults-Wilson, W. A., Reinsch, B. C., Tsyusko, O. V., Bertsch, P. M., Lowry, G. V., & Unrine, J. M. (2011b). Role of particle size and soil type in toxicity of silver nanoparticles to earthworms. Soil Science Society of America Journal, 75 , 365–377.
Silva, T., Pokhrel, L. R., Dubey, B., Tolaymat, T. M., Maier, K. J., & Liu, X. (2014). Particle size, surface charge and concentration dependent ecotoxicity of three organo-coated silver nanoparticles: comparison between general linear model-predicted and observed toxicity. Science of the Total Environment, 468 , 968–976.
Sotiriou, G. A., Meyer, A., Knijnenburg, J. T. N., Panke, S., & Pratsinis, S. E. (2012). Quantifying the origin of released Ag+ ions from nanosilver. Langmuir, 28 , 15929–15936.
Thuesombat, P., Hannongbua, S., Akasit, S., & Chadchawan, S. (2014). Effect of silver nanoparticles on rice (Oryza sativa L. cv. KDML 105) seed germination and seedling growth. Ecotoxicology and Environmental Safety, 104 , 302–309.
Tian, Y. (2010). Transport of engineered nanoparticles in saturated porous media. Journal of Nanoparticle Research, 12 , 2371–2380.
Wiechmann, B., Dienemann, C., Kabbe, C., Brandt, S., Vogel, I., & Roskoch, A. (2012). Klärschlammentsorgung in der Bundesrepublik Deutschland . Bonn: Umweltbundesamt.
Yin, Y., Yu, S., Shen, M., Liu, J., & Jiang, G. (2015a). Fate and transport of silver nanoparticles in the environment. Silver nanoparticles in the environment (pp. 73–108). Springer.
Yin Y., Yu S., Yang X., Liu J., & Jiang G. (2015b). Source and pathway of silver nanoparticles to the environment. Silver nanoparticles in the environment. Springer, 43–72.
Yu, S., Yin, Y., & Liu, J.-F. (2013). Silver nanoparticles in the environment. Environmental Science: Processes & Impacts, 15 , 78–92.
Yu, S., Zhou, X., & Liu, J. (2015). Separation and determination of silver nanoparticles. Silver nanoparticles in the environment (pp. 9–42). Springer.
Zhang, H., Smith, J. A., & Oyanedel-Craver, V. (2012). The effect of natural water conditions on the anti-bacterial performance and stability of silver nanoparticles capped with different polymers. Water Research, 46 , 691–699.
Download references
Acknowledgements
We would like to thank the Soil Physics and Land Management laboratory and RIKILT for their contribution and for the use of their facilities and devices for executing the experiments. Many thanks to Demie Moore for suggestions regarding the English editing.
This study received financial support from the Higher Committee for Education Development in Iraq.
Author information
Authors and affiliations.
Soil physics and land management group, Wageningen University and research, Wageningen, The Netherlands
Karrar N. M. Mahdi, Martine van der Ploeg, Coen Ritsema & Violette Geissen
College of Engineering, University of Baghdad, Baghdad, Iraq
Karrar N. M. Mahdi
RIKILT Wageningen University and Research, Wageningen, The Netherlands
Ruud Peters
You can also search for this author in PubMed Google Scholar
Corresponding author
Correspondence to Karrar N. M. Mahdi .
Rights and permissions
Open Access This article is distributed under the terms of the Creative Commons Attribution 4.0 International License (http://creativecommons.org/licenses/by/4.0/), which permits unrestricted use, distribution, and reproduction in any medium, provided you give appropriate credit to the original author(s) and the source, provide a link to the Creative Commons license, and indicate if changes were made.
Reprints and permissions
About this article
Mahdi, K.N.M., Peters, R., van der Ploeg, M. et al. Tracking the Transport of Silver Nanoparticles in Soil: a Saturated Column Experiment. Water Air Soil Pollut 229 , 334 (2018). https://doi.org/10.1007/s11270-018-3985-9
Download citation
Received : 12 June 2018
Accepted : 18 September 2018
Published : 01 October 2018
DOI : https://doi.org/10.1007/s11270-018-3985-9
Share this article
Anyone you share the following link with will be able to read this content:
Sorry, a shareable link is not currently available for this article.
Provided by the Springer Nature SharedIt content-sharing initiative
- Silver nanoparticles
- Find a journal
- Publish with us
- Track your research

IMAGES
VIDEO
COMMENTS
This paper provides a set of comprehensive guidelines for the use of dynamic column breakthrough experiments to measure single and multi-component equilibria and kinetics. Recommendations are made for the design of the experimental rig, experimental procedures, and data processing methods to minimize errors and increase data reliability. Designing experiments to identify the transport ...
Repeat the above experiment with two changes: (1) use 10 ppm Cu solution (remember that Cl is an interfering species for Cu electrode, so make sure that all Cl is flushed out of the system; use non-Cl background solution, e.g., Na2SO4); (2) do a pulse input instead of step input (create a pulse and trace it at the column outlet); the
Present paper involved the review of fixed-bed column studies for removal of various contaminants from synthetic wastewater. Basic concept of adsorption, its types (i.e., chemisorption and physisorption) and its mechanism, adsorbents and adsorbates were included. Comparison of batch and column adsorption study is mentioned. Complete study of breakthrough curve for designing adsorptive column ...
The most common approach to study solute transport on the continuum scale is the column outflow experiment [Lewis and Sjöstrom, 2010]. Usually, column experiments are run in an open-flow mode. Thereby, the influent solution is fed to the column and the effluent is collected by, e.g., a fraction collector and analyzed for target compounds.
Details of the column experiment setup including column material, fittings, tubes, pumps, and column size were reviewed by Banzhaf and Hebig ... Generally, the experiments were carried out up to about 100 effluent pore volumes, equivalent to up to 20-35 L. Table 4. Characteristics of filter materials (FMs) based on iron (Fe), calcium (Ca) or ...
Column effluent compositions during initial phases of the column experiments were previously described (White et al., 1999a, White et al., 1999b, White and Brantley, 2003). Data collected at longer times (4.0-13.8 yrs) are the focus of the present paper and are summarized in Table 5 (see Supplemental tables for the complete data sets).
A widely used approach for the determination of various transport parameters is the application of laboratory scale column outflow experiments [Lewis and Sjöstrom, 2010], where the main observation is the time-dependent column effluent solution, frequently called breakthrough curve (BTC). The information on the processes that govern transport ...
Sampling the effluent or soil solution in such a way that the column remains unsaturated is challenging. ... However, the hydraulic conductivity of a soil at or near 1013 mbar matric potential is so low that any column experiment would take a prohibitively long time (Wilson et al., 1995). As a result, most unsaturated soil column studies are ...
The breakthrough curve for NH 4 + through the sand column during the wet and dry periods over a 4 day column experiment with an initial concentration of 35 mg/L is shown in Fig. 1—NH 4.
Moreover, Daus et al. 19 found that the sorption capacity of As(V) when using GIH in column experiments (2.3 mg/g) was 2.3 times lower than that in batch experiment (5.2 mg/g) due to preferential ...
Column experiments are fast, flexible, and easy to man-age; their boundary conditions can be controlled and they are cheap compared to extensive field experiments. They can provide good estimates of all relevant transport parameters. However, the obtained results will almost always be limited to the scale of the experiment and are not ...
Early in the experiment, concentrations of As rose in the effluent from the column (Fig. 1).This was accompanied by <5% reduction of Fe(III) oxides to Fe(II) in the aquifer sediment over the ...
The unit sorption obtained during 95 days of column experiment was 10.668, 4.277, and 2.286 mg P-PO4 g⁻¹ for 1.0, 2.5, and 5.0%, respectively. For practical implementation, the most recommended ...
The column-experiment samples were analyzed by LCMS and by the methylene blue active substances (MBAS) assay. An overview of the analytical methods is provided in the SI ... Solute concentrations measured for column-effluent samples were used to develop breakthrough curves (BTC) as a function of pore volumes eluted, with pore volumes ...
Isotopes and the soil column experiment provide insight into the potential impact of soil disturbance on SO 4 concentrations in water. The SO 4, δ 34 S-SO 4, and δ 18 O-SO 4 values of influent water and effluent water samples in the soil column revealed the influence of disturbed soil on water quality. In the natural soil, evaporites exist in ...
The column experiment was conducted and modeled through a developed numerical model ... some regions, treated wastewater effluent from the wastewater treatment plant (WWTP)
Column Chromatography - Column chromatography is a technique which is used to separate a single chemical compound from a mixture dissolved in a fluid. ... Before starting with the Column Chromatography Experiment let us understand the different phases involved. ... and collecting the components in separate effluent fractions. The best ...
As a conclusion based on these theoretical considerations column tests (a) equilibrate much faster than batch leaching tests and (b) the equilibrium concentrations are maintained in the column effluent even for slow intraparticle diffusion limited desorption for extended periods of time (>days).
In most soil column experiments, the objective of the initial conditions is to reproduce the prevailing environmental conditions — specifically the flow rate of pore water and moisture content profile if the experiment is unsaturated. Since most column studies are performed at steady-state rather than under transient conditions, the initial ...
If the curves in this figure represent effluent concentrations from a tracer experiment on a chromatographic column, the number of plates is defined as: Figure 1. A Gaussian Distribution and the height of a plate, H is: The vertical scale, corresponding to effluent concentration in our situation, does not affect calculation of H or N.
In the second stage (SD), the decrease was 62.8%, resulting in a final effluent with 67.7 mg/L COD, which corresponded to a global COD decrease of 97.4%. For the toxicity tests, radish seeds were ...
Bottlenecks inherent in batch and column adsorption configurations have impeded the implementation of the adsorption technique in large-scale wastewater treatment systems. This study mainly aimed to develop an innovative wastewater treatment prototype that integrates inclined plate settlers (IPS) and composite adsorbent coating (CAC). The objective is to enable the removal of Cd2+ from aqueous ...
Based on the soil column experiment, this study analyzed the concentrations of sulfate (SO 4), sulfur, and oxygen isotopic composition of SO 4 (δ 34 S-SO 4 and δ 18 O-SO 4) in effluent water. The water-rock interaction mechanisms in the disturbed soil and the contribution of this interaction to the SO 4 in groundwater were studied.
During the experiment, the collection of the effluent water (EW) containing leached AgNPs was started after the addition of each RW pulse to a column and continued for 24 h. For column series 1 (C24), only 1 volume of EW was collected. For column series 2 (C48), 2 EW volumes were collected and for column series 3 (C72), 3 were collected.