
An official website of the United States government
The .gov means it’s official. Federal government websites often end in .gov or .mil. Before sharing sensitive information, make sure you’re on a federal government site.
The site is secure. The https:// ensures that you are connecting to the official website and that any information you provide is encrypted and transmitted securely.
- Publications
- Account settings
- My Bibliography
- Collections
- Citation manager

Save citation to file
Email citation, add to collections.
- Create a new collection
- Add to an existing collection
Add to My Bibliography
Your saved search, create a file for external citation management software, your rss feed.
- Search in PubMed
- Search in NLM Catalog
- Add to Search
Morphological differences in human zygotes and embryos cultured in different media
Affiliation.
- 1 Campinas Center of Human Reproduction, Campinas, São Paulo, Brazil.
- PMID: 22126911
- DOI: 10.1017/S0967199411000670
Purpose: To compare the effects of four culture media on the quality of human zygotes and embryos.
Methods: Prospective study analyzing 2289 human embryos cultivated simultaneously in two different culture media: HTF, the default medium, with either Universal IVF, Global or IVF-30 as the secondary media. The sibling oocytes by each patient were randomly divided between the two culture media following intracytoplasmic sperm injection (ICSI). On day 1 the pronuclear stage of zygotes were evaluated and on day 2 embryos were evaluated according to the number of cells, percentage of fragmentation and number of nuclei. Z-test and odds ratios were used in the statistical analysis.
Results: There was a higher percentage (55.2%) of class A1 + A2 zygotes with IVF-30 compared with HTF, Global or Universal IVF media (49.1%, 44.7% and 44.2%, respectively). The percentage of Top embryos was significantly higher with Global (40.2%) compared with HTF (21.3%), IVF-30 (25.0%) or Universal IVF media (11.2%).
Conclusions: Global medium produced more Top embryos evaluated on day 2 of development.
PubMed Disclaimer
Similar articles
- [Embryo development in two single-step media: Analysis of 2059 sibling oocytes]. Durand M, Sermondade N, Herbemont C, Benard J, Gronier H, Boujenah J, Cédrin-Durnerin I, Poncelet C, Grynberg M, Sifer C. Durand M, et al. Gynecol Obstet Fertil. 2016 Mar;44(3):163-7. doi: 10.1016/j.gyobfe.2016.01.005. Epub 2016 Feb 18. Gynecol Obstet Fertil. 2016. PMID: 26908149 French.
- Changes in histone methylation during human oocyte maturation and IVF- or ICSI-derived embryo development. Qiao J, Chen Y, Yan LY, Yan J, Liu P, Sun QY. Qiao J, et al. Fertil Steril. 2010 Mar 15;93(5):1628-36. doi: 10.1016/j.fertnstert.2009.03.002. Epub 2009 Apr 25. Fertil Steril. 2010. PMID: 19394606
- Comparison between intracytoplasmic sperm injection and in vitro fertilisation employing oocytes derived from prepubertal goats. Jiménez-Macedo AR, Izquierdo D, Anguita B, Paramio MT. Jiménez-Macedo AR, et al. Theriogenology. 2005 Oct 1;64(6):1249-62. doi: 10.1016/j.theriogenology.2004.11.025. Epub 2005 Mar 29. Theriogenology. 2005. PMID: 16139602
- Can Nucleoli Be Markers of Developmental Potential in Human Zygotes? Fulka H, Kyogoku H, Zatsepina O, Langerova A, Fulka J Jr. Fulka H, et al. Trends Mol Med. 2015 Nov;21(11):663-672. doi: 10.1016/j.molmed.2015.09.005. Epub 2015 Oct 20. Trends Mol Med. 2015. PMID: 26494190 Review.
- Is there an optimal pH for culture media used in clinical IVF? Swain JE. Swain JE. Hum Reprod Update. 2012 May-Jun;18(3):333-9. doi: 10.1093/humupd/dmr053. Epub 2012 Feb 6. Hum Reprod Update. 2012. PMID: 22311857 Review.
- Culture media for human pre-implantation embryos in assisted reproductive technology cycles. Youssef MM, Mantikou E, van Wely M, Van der Veen F, Al-Inany HG, Repping S, Mastenbroek S. Youssef MM, et al. Cochrane Database Syst Rev. 2015 Nov 20;2015(11):CD007876. doi: 10.1002/14651858.CD007876.pub2. Cochrane Database Syst Rev. 2015. PMID: 26585317 Free PMC article. Review.
- Search in MeSH
LinkOut - more resources
Full text sources.
- Cambridge University Press
- Citation Manager
NCBI Literature Resources
MeSH PMC Bookshelf Disclaimer
The PubMed wordmark and PubMed logo are registered trademarks of the U.S. Department of Health and Human Services (HHS). Unauthorized use of these marks is strictly prohibited.

An official website of the United States government
The .gov means it’s official. Federal government websites often end in .gov or .mil. Before sharing sensitive information, make sure you’re on a federal government site.
The site is secure. The https:// ensures that you are connecting to the official website and that any information you provide is encrypted and transmitted securely.
- Publications
- Account settings
Preview improvements coming to the PMC website in October 2024. Learn More or Try it out now .
- Advanced Search
- Journal List
- Int J Mol Med
The molecular basis of fertilization (Review)
Katerina georgadaki.
1 Institute of Biology, Medical Chemistry and Biotechnology, National Hellenic Research Foundation, Athens 116 35
Nikolas Khoury
Demetrios a. spandidos.
2 Laboratory of Clinical Virology, School of Medicine, University of Crete, Heraklion 71003, Greece
Vasilis Zoumpourlis
Fertilization is the fusion of the male and female gamete. The process involves the fusion of an oocyte with a sperm, creating a single diploid cell, the zygote, from which a new individual organism will develop. The elucidation of the molecular mechanisms of fertilization has fascinated researchers for many years. In this review, we focus on this intriguing process at the molecular level. Several molecules have been identified to play a key role in each step of this intriguing process (the sperm attraction from the oocyte, the sperm maturation, the sperm and oocyte fusion and the two gamete pronuclei fusion leading to the zygote). Understanding the molecular mechanisms of the cell-cell interactions will provide a better understanding of the causes of fertility issues due to fertilization defects.
1. Introduction
Fertilization is a sequence of coordinated molecular events involving the merging of the sperm with the egg, the fusion of the pronuclei and the intermingling of the maternal and paternal chromosomes. The first form of human life is the zygote (a diploid cell) from which the new organism will result. During sexual intercourse, millions of sperm are deposited into the vagina. A number of these will die in the acidic environment. However, many will survive due to the protective elements provided in the fluids surrounding them. Soon afterwards, the sperm have to swim through the cervical mucus, towards to the uterus and then on to the fallopian tubes. As they swim towards these, they decrease in number, in an attempt to make it through the mucus. Inside the uterus, the contractions of the uterus assist the journey of the sperm towards the egg. Fertilization takes place in the ampulla of the oviduct. If the oocyte is not fertilized here, it slowly passes to the uterus, where it becomes degenerated and is absorbed. Achieving fertilization requires the activation of sperm and oocyte maturation. However, the oocyte/sperm interaction depends on a number of changes occurring in the egg and the sperm.
The egg complex following ovulation enters the oviduct consisting of three components: i) the oocyte (egg) arrested at metaphase of meiosis II; ii) the extracellular matrix of the egg or zona pellucida consisting of three glycoproteins (ZP1, ZP2 and ZP3), synthesized and secreted by the oocyte; iii) the cumulus oophorus, consisting of granulosa cells enriched with hyaluronic acid ( 1 , 2 ). Cumulus cells support fertilization, and in vitro fertilization can be achieved more efficiently with them than without them ( 3 – 5 ).
The journey of the sperm is facilitated by ovarian hormones that affect the structure, composition and activity of the secretory epithelia of the cervix, the uterus and the fallopian tubes and the contractility of these elements. The estrogen hormones, favor these factors, while progesterone does not. Oxytocin, which is secreted during intercourse by stimulation of the posterior pituitary, causes the contraction of the uterus and the fallopian tubes, as well as by the prostaglandins that affect the contractility of the uterus and fallopian tubes. During the ovulation period, the uterus becomes more sensitive to prostaglandins.
Oocytes acquire the ability to fuse with sperm when they reach 20 µ m in diameter and they are arrested at the prophase of meiosis II ( 6 ). Spermatozoa undergo a series of events during maturation in order to acquire motility and fertility, as they move from the proximal towards the distal end of the epididymis. Only spermatozoa that have passed through the epididymis are mature enough to be capable of motility. Moreover, via deposition of new proteins in the nucleus, the DNA becomes more condensed. The sperm head decreases in size and becomes more compact. The above is crucial for the subsequent correct decondensation of the paternal DNA in the maternal oocyte. The ability for motility is achieved, but at the same time is inhibited by the milieu. Finally, the structure of the plasma membrane is altered by the addition of glycoproteins and other proteins. This affects the motility, the capacitation ability and the ability for the acrosome reaction ( http://www.embryology.ch/anglais/dbefruchtung/bereitstell02.html#genitaltrakt ).
Sperm is the male gamete and is derived from the Greek word 'sperma' (meaning 'seed'). It consists of three parts, a head (containing DNA and enzymes in order to penetrate the oocyte), a midpiece (containing cellular elements, centrioles, microtubules and a number of mitochondria for energy production required to promote sperm) and a tail (enabling sperm movement) ( http://www.newworldencyclopedia.org/entry/Sperm ).
The head of normal sperm has an oval shape, a length of 3–5 µ m, a width of 2–3 µ m and is 1.5 µ m thick. It consists of the nucleus and the acrosome, which are covered by the nuclear membrane and the metacrosomic sheath. The nucleus contains the male genetic material. The DNA of the sperm has a slightly different structure from the DNA of a diploid (somatic) cell, meaning that the DNA is concentrated in the smallest possible volume, so as to save space. The acrosome contains proteolytic enzymes, such as acrosin, trypsin, hyaluronidase and proteases, which are released during the acrosome reaction and hydrolysis in granular cells and the zona pellucida of the egg in order to assist the penetration of the sperm and the merger with the egg ( 7 ).
The neck or mid-section of the sperm connects the head to the tail (length, 7.5 µ m; and width, 1 mm). It contains the axial filament surrounded by fibrils with circular layout and one to two centrosomes. The neck contains mitochondria, enzymes of glycolysis and oxidation systems and provides the necessary energy for survival and mobility.
The tail (length, 40–50 mm) consists of a total of ten pairs of fibrils (a central and nine peripheral) and is responsible for the typical sperm motility.
The sperm can survive for 3–5 days inside the vagina, particularly in the vagina or mucous membranes in the upper part of the female genital tract. Fertilization in this case is possible if the sperm remain alive. A single sperm is sufficient to fertilize the egg. Sperm survive outside the female body for up to several hours. When the sperm meets the oocyte, they swim around it. Subsequently, one of them adheres to the head of the oocyte and begins rotational movements about the longitudinal axis. The whole sperm then penetrates the oocyte, with the acrosome penetrating the egg cover [ http://www.yourarticlelibrary.com/biology/human-reproduction/structure-of-human-sex-gametes-spermatozoan-and-ovum-biology/26913/ ; http://www.onmed.gr/ygeia/story/329099/poso-zei-to-sperma-mesa-ston-kolpo#ixzz44lwgUQgb (in Greek)].
2. Molecules synthesized and secreted from the oocyte, which orient and stimulate sperm
Resact (respiratory activating peptide) is a peptide of 14 amino acids, isolated from the egg jelly of the sea urchin, Arbacia punctulata ( 8 ). It is probably recognized by the guanylate cyclase (guanylate cyclase), a protein of the cell membrane of the sperm, which causes an increase in Ca 2+ levels. Experimentally, when resact is added to a solution with spermatozoa, the latter undergoes helical movements directed towards the point of resact ( http://worms.zoology.wisc.edu/dd2/echino/fert/chemo-taxis/chemotaxis.html ).
The sperm receptor for resact is a transmembrane protein. Once it binds resact on the extracellular side, it causes a conformational change on the cytoplasmic side, and it activates the receptor's enzymatic activity. This triggers the mitochondrial ATP-generating apparatus, as well as the dynein ATPase that stimulates flagellar movement in the sperm ( 9 , 10 ).
Speract (sperm activating peptide) consists of ten amino acids and appears to promote the increase in pH and promotes sperm movement. The speract receptor is localized to the sperm tail ( 11 ), which contains the axoneme, the organelle of motility. The signaling in the sperm tail is central to motility regulation. There is evidence that speract can modulate sperm motility ( 12 ).
3. The three stages of fertilization
Sperm preparation: capacitation and acrosome reaction.
The phase of sperm maturation is known as activation (sperm capacitation). It occurs in the genital tract of the female, and acts as a preparatory step for the acrosome reaction. Activation does not include morphological changes and is accompanied by hyperactivation of the sperm which is a strong, non-linear motion ( 2 ). An important role is played by proteolytic enzymes ( 13 ).
In mammals, the ejaculated sperm is motile. However, their ability to fertilize an oocyte is reduced. The latter may occur after removing inhibitory factors, such as surface-attached glycoproteins, seminal plasma proteins and the depletion of membrane cholesterol. This final state of activated sperm is known as hyperactivation, and is a high energy phase of vigorous flagellar movement and swimming capacity ( 14 ).
Capacitation involves a number of processes, such as functional coupling of the signal transduction pathways that regulate the initiation of acrosome reactions by ZP3; alterations in flagellar motility that may be required to penetrate the zona pellucida; and the development of the capacity to fuse with eggs ( 15 ). The above-mentioned events are followed by alterations in metabolism, membrane biophysical characteristics, changes in the protein phosphorylation state, elevations in intracellular pH and calcium levels, and hyperpolarization of membrane potential. In vivo , many factors are likely to mediate the activation of sperm. Sterol binding proteins, such as high density lipoprotein, have been identified in the fallopian tubes and can accelerate the efflux of cholesterol from the sperm ( 16 ). Furthermore, progesterone can regulate some aspects of sperm activation. It is present in the environment of the fallopian tubes, derived both from follicular fluid and cells of the cumulus oophorus ( 17 ). Glucose is known to be essential for successful capacitation. It functions not only as an energy molecule allowing spermatozoa to swim, but it also enables the spermatozoa to fertilize eggs ( 18 ).
The agents of the oocyte mucous that cause the acrosome reaction are specific for each species. In mammals, sperm recognizes and binds to ZP3 glycoprotein of the zona pellucida. The O-polysaccharide connections to the core protein of ZP3 seem to be necessary in this step. It is believed that the terminal galactose of O-linked oligosaccharides reacts with galactosyltransferase (Mr 56 kDa), a cell membrane protein in the anterior end of the sperm head. The acrosome reaction of the sperm is caused by the core protein of ZP3. Once the core protein of ZP3 is damaged, this reaction is inhibited, but not the binding of the sperm to the zona pellucida. The sperm reaching the transparent zone is connected via SED1 protein to ZP3. As a result of irreversible binding of the sperm to the egg, the zona pellucida triggers the acrosome reaction.
The outer plasma membrane of the acrosome fuses at multiple sites with the plasma membrane and the contents of the acrosome are released. After the acrosome reaction, it is believed that the protein pre-acrosin of the acrosomal vesicle binds to ZP2, and becomes activated, forming the enzyme acrosin which digests the transparent area at this point. Digestion of the zona pellucida is followed by the fusion of the membrane of the sperm to the egg membrane, which seems to be caused by the PH-30 sperm protein ( 19 ).
PH-30 is a transmembrane glycoprotein consisting of an α and β subunit. The α subunit (289 amino acids) contains a sequence between 90 and 111 which has many similarities to the 'fusion peptide' E2 glycoprotein of the rubella virus. The β subunit (353 amino acids) contains the tripeptide RGD (arginine-glycine-aspartic acid) in the last 90 amino acids of the N-terminus. The tripeptide RGD is the active site which can be recognized by integrins of the cell membrane of the egg. This recognition is followed by the fusion of the membranes, which is caused by the α subunit of PH-30. Both subunits derive from precursors that undergo proteolytic modification during the differentiation of sperm in the testes and their maturation in the epididymis. PH-30 is located in the cell membrane in the anterior portion of the side part of the mature sperm head ( 19 , 20 ). The acrosome reaction involves the fusion of the acrosome membrane with the overlying plasma membrane of the sperm and vesicle formation. The result is the release of peptidases from the acrosomal vesicle that digest the mucous coat. The fusion of the membranes appears to be caused by the entry of Ca +2 ions and the exit of K + ions. The sperm plasma membrane that does not participate in the acrosome reaction and overlying the acrosome is known as the equatorial region. This seems to be the site of sperm and egg fusion ( 2 ). An acrosomal filament, is formed by the polymerization of G (Globular) actin to F (Fibrilar) actin. Desmin (30.5 kDa) is exposed to the acrosomal filament. The polymerization of actin is induced by the increase in pH (~7.4), which results from the input of Na + and H + output. The activation of dynein ATPase and the use of ATP results in an increased movement of the sperm. cAMP levels increase (approximately 400-fold) in the cytoplasm of sperm. This increase marks the beginning of the process of chromatin decondensation of the sperm, before the formation of the zygote nucleus.
P4 (progesterone) is a well-studied molecule involved in the modulation of sperm function. Its role in sperm capacitation has been revealed; however, the exact mechanisms involved are not yet fully understood. Progesterone is involved not only in capacitation, but also in other events, such as hyperactivated motility, flagellar bending, in chemotaxis, in acrosome reaction and in sperm-ZP binding and sperm-oocyte fusion. Its effects on sperm function have been studied in humans, as well as in other mammals (mouse, boar) ( 21 – 24 ).
Evans and Florman, in Nature Cell Biology and Nature Medicine ( 15 ), propose a model according to which in the sperm head, the receptor TPC2-ZP3 is triggered by its association with the zona pellucida. This results in calcium entry through T-type channels, leading to a transient increase in calcium levels in the cytoplasm and the activation of phospholipase C (PLC) via protein G. PLC is divided into IP3 and DAG. This causes Ca + entrance and leads to the acrosome reaction.
In the capacitation process, phosphatidyl-inositol-3-kinase (PI3K) is phosphorylated/activated via a protein kinase A (PKA)-dependent cascade, and is downregulated by PKCa. At the beginning of capacitation, PKCa is active and results in PI3K inactivation. PKCa and PP1c2 are degraded by a PKA-dependent mechanism, and this allows the activation of PI3K. The activation of PKA, which depends on cAMP produced by the bicarbonate-dependent soluble adenylyl cyclase, leads to an increase in actin polymerization, an essential step so as to reach hyperactivated motility, which is necessary for successful fertilization ( 25 ).
The sperm-specific CatSper ion channels have been suggested to control the intracellular Ca 2+ concentration and, thereby, the swimming behavior of spermatozoa ( 26 ). The CatSper channel seems to be activated by progesterone in human spermatozoa ( 29 ), which is interesting from the sperm-egg interaction viewpoint, as cumulus cells are known to produce progesterone ( 5 ).
Sperm-egg binding and fusion
The plasma membrane of the oocyte consists of two sites, a microvilli-free region and a microvilli-rich region. The fusion of sperm and oocyte takes place in the microvilli-rich region ( 28 , 29 ). The interaction between sperm and oocyte is preceded by acrosomal exocytosis, triggered by sperm and ZP binding. It involves sperm attachment to the oocyte and cell-cell adhesion, leading to membrane fusion of the two gametes ( 30 ). The inner acrosomal membrane of the sperm, being exposed following the acrosome reaction, comes into contact with the oocyte membrane ( 31 ). The equatorial segment adheres to the posterior head of the sperm and fuses with the oocyte membrane ( 32 , 33 ).
Several molecules have been identified in sperm and oocytes, with a crucial role in gamete binding. Fertilin α, fertilin β and cyritestin are also known as ADAM1, ADAM2 and ADAM3, respectively. Members of this family consist of a signal sequence domain, a metalloprotease domain, a disintegrin-like domain, a cysteine-rich domain and an epidermal growth factor-like repeat. A number of studies have revealed a role of ADAM1, ADAM2 and ADAM3 in sperm oocyte binding ( 30 ). Fertilin β has been shown not to be essential for plasma membrane binding and fusion. Studies on fertilin β and cyritestin with knockout mice have shown a reduced adhesion to the oocyte plasma membrane; however, some sperm adhere and they fuse with the oocyte ( 15 , 34 ). Moreover, they show poor adhesion pattern to the ZP ( 34 – 36 ).
As regards oocytes, integrins found on the egg surface are thought to be receptors for sperm ADAMs. Studies have revealed that α6β1 integrin is an egg receptor for fertilin β ( 37 – 39 ). Other studies have indicated that α9β1 integrin is a receptor for fertilin β ( 40 , 41 ) CD46 has been found to be expressed in rodents on the acrosomal membrane of sperm ( 42 ). CD46 interacts directly with β1 integrin and indirectly with tetraspanins in human cells ( 43 ). However, the key role of CD46 appears to stabilize the acrosomal membrane ( 44 ).
As an integrin-associated protein, in the oocyte, CD9 is essential for sperm-egg interactions. It is a member of a tetraspanin protein family expressed on the mouse egg surface ( 45 ). The role of CD9 in sperm oocyte fusion has been demonstrated in a number of studies with CD9-null oocytes which showed reduced ability for strong sperm adhesion ( 46 ). CD9-deficient mice show reduced fertility ( 47 – 49 ). Another tetraspanin member, CD81, is expressed on the oocyte surface and interacts with CD9 ( 50 ). Deletion of the CD81 gene results in a reduction in fertility. However, its role in sperm oocyte interaction has not yet been fully elucidated ( 51 , 52 ).
CRISP1, a sperm protein expressed by the cumulus cells surrounding the oocyte, stimulates sperm orientation through the modulation of sperm hyperactivation and it seems to regulate CatSper ( 53 ). Recent studies have revealed an oocyte-derived chemotactic activity associated with a hydrophobic non-peptide molecule in human sperm ( 54 ) and an association between follicle rupture and uterine contractions with the success of human in vivo insemination, suggesting the existence of possible chemoattractive substances in the female tract ( 55 ). It is not clear if there is a binding partner on the oocyte membrane for sperm-associated CRISP protein, along with the exact mechanisms through which gamete binding is achieved.
The sperm-specific protein, Izumo, is essential for sperm-egg plasma membrane binding and fusion ( 56 ). IZUMO interacts directly with some molecules on the oolema. On the oocyte side, Juno is a member of the folate receptor family and recognizes the sperm IZUMO, facilitating fertilization. It has been revealed that mice lacking Juno on the surface of their egg cells are infertile as their egg cells do not fuse with normal sperm. This finding demonstrates the essential role of Juno in the fertility of female mice ( 57 ). Other studies have shown that a helical dimer of fragments of the N-terminus domain of IZUMO is required for sperm-oocyte fusion ( 58 ). IZUMO forms complexes with other proteins on the sperm surface via its N-terminal domain which forms dimers ( 56 ). Therefore, IZUMO plays a key role in organizing and stabilizing a protein-like complex crucial for membrane fusion. Inoue et al ( 56 ) found a protein, angiotensin-converting enzyme 3 (ACE3) on the sperm acrosomal cap capable of interacting with IZUMO ( 59 ).
Studies have revealed the rapid loss of Juno from the oocyte membrane soon after fertilization. This implies that Juno is essential for the process of fertilization, thus, being the basis for polyspermy block in mammals. One possible explanation for this process is that Juno is shed in vesicles following fertilization, generating a zone of 'decoy oocyte' confined within the perivitelline space that could bind acrosome-reacted sperm and therefore avoid supernumerary sperm entry ( 60 ). Although it has been validated that Juno protein as the first cell surface receptor conserved in mammals, the interaction between IZUMO1 and Juno seems to be a necessary and an essential adhesion step; however, its role in the gamete fusion mechanism is not yet clear ( 57 ).
Two molecules, trypsin-like acrosin and spermosin proteases have been proposed to be involved in the first physical contact of the two gametes, suggesting that a proteasome system enables sperm to penetrate the chorion or participate in the process as sperm-related 'moveable' binding proteins ( 52 ). Sperm hyaluronidases are believed to play an intriguing role in fertilization in mammals, and sperm-specific SPAM1 and HYAL5 hyaluronidase have been suggested to be involved in sperm-ZP binding in mice. Recent studies have shown that hyaluronidases are not required for fertilization ( 61 , 62 ).
It is important to mention that the oviductal environment and its secretions also play an essential role in the transport and interaction of male and female gametes. The expression of lactoferrin, a human oviductal protein, is able to inhibit gamete interaction in vitro and it seems to be involved in the regulation of the reproductive process through a role in polyspermy prevention ( 63 ). It has also been shown that lactoferrin causes a decrease in sperm α-D-mannose binding sites and an increase in tyrosine phosphorylation of sperm proteins, thus implying that this protein is able to modulate the parameters of sperm function ( 64 ).
Cortical reaction: Meiosis resumption of oocyte and activation of the zygote
Once the sperm fuses with the oocyte, the beating of the tail stops immediately. The fusion of sperm and the oocyte membrane appears to cause the polymerization of actin and microvilli extension. The sperm instead is drawn into the oocyte by elongation and fusion of the microvilli of the egg. As a result, the sperm nucleus and other organelles are incorporated into the oocyte cytoplasm. The actin filaments are essential for the attraction of the sperm into the oocyte. The cytoplasm swells and forms colliculus (~7 microns in length and 2 microns wide) resembling a so-called fertilization cone. In some invertebrates and amphibians, there are certain areas of the oocyte membrane for the binding and fusion of the sperm.
Seconds after fertilization, the membrane potential of the oocyte undergoes a large depolarization via a massive influx of Na + ions. The depolarization of the egg takes about a minute to repolarize via K + leakage. This is the fast block to polyspermy: sperm cannot fuse to a membrane that is not −70 mV ( https://structureandfunction.wordpress.com/2013/02/28/the-fast-and-slow-blocks-to-polyspermy-and-egg-activation/ ).
CG distribution and the cortical reaction following calcium oscillation are crucial steps in the prevention of polyspermy. Calcium released from the endoplasmic reticulum is dependent on the quality and quantity of the mitochondria, which are markers of oocyte quality, since a low mitochondrial DNA copy number results in poor oocyte developmental competence ( 65 , 66 ).
PLCζ from spermatozoa is considered to be the responsible activator during this process ( 67 ); however, other factors may also be involved ( 5 , 68 ).
The cortical reaction is a process through which cortical granules from the oocyte are released preventing polyspermy. The fast block of polyspermy immediately prevents additional sperm getting attached to the oocyte. On the other hand, the cortical reaction establishes a permanent barrier to sperm entry and functions as the main part of the slow block of polyspermy in many animals. The cortical reaction is propagated over the surface of the egg by a wave of Ca ++ .
In this process, secretory vesicles located in the region below the plasma membrane of the oocyte, are fused with the oocyte plasma membrane. This results in the release of contents of the cortical granules, modifying the extracellular matrix so as to be impenetrable to other sperm. The cortical granules contain hydrolytic enzymes, such as proteases that clip perivitelline proteins, peroxidases that harden the vitelline envelope and glycosaminoglycans that attract water into the perivitelline space, causing it to expand and form the hyaline layer. Calcium ions from the cortical smooth endoplasmic reticulum are responsible for cortical granule rupture. It is believed that this is provoked by the activation of a G-protein in the plasma membrane of the oocyte. The sperm binds to ZP3 which is consistent with the G-protein and becomes active. Bindin binds the sperm to the oocyte cell membrane, but another sperm factor activates a receptor, which in turn activates the G-protein. G-proteins of the spermatozoon itself (activated when sperm was in contact with the mucous of the egg shell with the initiation of the acrosome reaction) activate enzymes, which results in increased Ca +2 levels in the egg.
In mammals, the cortical reaction modifies the zona pellucida, leading to the block of polyspermy. Several enzymes are released by the cortical granules, leading to the digestion of the sperm receptor glycoproteins ZP2 and ZP3, so that they can no longer bind spermatozoon ( https://en.wikipedia.org/wiki/Cortical_reaction ).
It is interesting to know hows sperm factor causes the release of Ca +2 . Activated G-protein activates the enzyme, PLC. The latter cleaves the lipid phosphotidylinositol 4,5-biphosphate (PIP2) in diacyglycerol (DAG) and inositol 1,4,5-triphosphate (IP3). PIP2 and DAG are second messengers. IP3 binds to its receptors in the endoplasmic reticulum, at the entry point of the sperm. This results in the release of Ca +2 from the endoplasmic reticulum at this point. Ca +2 ions bind to sensitive Ca +2 receptors in the endoplasmic reticulum around the cortical granules. Ca +2 binding to these receptors results in the release of more Ca +2 , which spreads like a wave in the region (cortex) of the egg and causes the rupture of cortical granules. DAG activates PKC which phosphorylates protein exchange ions Na + to H + . The protein that exchanges ions, is activated by Ca +2 and the result of this activation is the entrance to Na + and H + output and an increase in pH from 6.8 to 7.3 in the egg. This 'wakes up' the oocyte from the metabolic inertia ( 69 ).
The last phase of oocyte activation is the resumption and completion of meiosis. This leads to polar body extrusion, cleavage of the zygote and embryonic cell divisions. There are widespread changes in molecules, such as proteins and RNAs that are not necessarily involved in meiosis resumption. Changes in proteomes and their composition have been revealed, showing significant degradation of maternal proteins. This is due to protein degradation, phosphorylation, post-translational modifications and new translation of maternal RNAs ( 70 ).
Cytoplasmic polyadenylation involves the elongation of the poly(A) tail after the export of mRNAs to the cytoplasm. It has been observed and described in the oocytes and early embryos of many animal species, from invertebrates to mammals, and is universally considered to be a regulatory mechanism for protein expression from specific mRNAs. The mediators of this process (cytoplasmic polyadenylation elements and their binding proteins) have been described in detail and some new findings have been reviewed recently ( 71 , 72 ).
The sperm nucleus undergoes a series of changes, including chromatin decondensation and the formation of a new nuclear envelope, to form the male pronucleus. The latter uses microtubules to migrate to the center of the cell, where it fuses with the female pronucleus to form a diploid nucleus. Other sperm organelles (e.g., mitochondria) persist during the early cleavage stages of the embryo and they may play a role in development ( 19 ).
The beginning, condensation of chromatin seems to happen with two histone phosphorylations of the sperm and their exchange with histones of the oocyte. Chromatin is condensed following interaction with histones H3 and H4, and X and Y sperm-specific proteins. In the cytoplasm of the ovum is the protein nycleoplasmin connected with histones H2A and H2B. As nucleoplasmin has greater affinity for the X and Y sperm proteins, the exchange of histones H2A/H2B with X and Y proteins occurs. This exchange allows the de-concentration and sharpness of the chromatin nucleus of the sperm. The process of decompression begins from the periphery to the center of the nucleus. When this process is finished, the vesicles of the nuclear membrane of the sperm bind to vesicles from the endoplasmic reticulum of the egg. The membrane of the pronucleus of the sperm is produced. While the pro nucleus of the sperm is formed, the nucleus of the oocyte completes the second division of meiosis and eliminates the second polar body. After entering the oocyte, the nucleus and the sperm centrosome rotate 180°, so that the centrosome is positioned between the nucleus of the sperm and ovum. The centrosome organizes microtubules that attach and pull the pronucleus of the sperm and the egg. Both pronuclei migrate towards each other and they are directed towards the center of the cytoplasm of the ovum. The membranes of the two pronuclei rupture and mixing of nuclear material is observed, resulting in the formation of the diploid nucleus of the zygote. The fusion of the pronuclei occurs in <1 h e.g., in Echinus (the meiotic division is finished before ovulation). Approximately 12 h in mammals including most eggs (e.g., human) is needed to complete the second division of meiosis with the excretion of the second polar body ( 73 ). Fig. 1 illustrates the events taking place in fertilization.

The events taking place in fertilization. (A) Sperm preparation-capacitation: Molecules (resact, speract) secreted from the oocyte, orient and stimulate sperm (guanylate cyclase). (B) Acrosome reaction: release of hydrolytic enzymes. The sperm via SED1 protein is connected to ZP3. (C) Fusion of sperm with plasma membrane of the oocyte: sperm pre-acrosin binds to ZP2. Proteins of sperm IZUMO, ADAMs 1, ADAMs 2, ADAMs 3 and CRISP1 bind to receptors on the oocyte (Juno, integrins, CD9, CD81). Other molecules identified playing role in gamete fusion are: Trypsin-like acrosin, spermosin, SPAM1, HYAL5, ACE3. (D) Cortical Reaction: Ca +2 release/wave of Ca +2 and formation of fertilization cone. Enzymes released by cortical granules, digest sperm receptors ZP2 and ZP3 (block of polyspermy). (E) Sperm chromatin decondensation to form male pronucleus: The oocyte nucleus completes the 2nd meiosis and eliminates the 2nd polar body.
4. Reorganization and partition of the zygote
Once the sperm enters the oocyte, the cytoplasmic material is reorganized. The cytoplasm of the oocyte contains cytoplasmic determinants that become distributed on different cells during cleavage. The cytoplasmic determinants (e.g., Vg1 and bicoid) directly or indirectly activate or inactivate genes and control events of differentiation in an early embryo. The point through which sperm has penetrated the oocyte marks the bilateral symmetry of the future adult organism. Following fertilization, cortical rotation occurs. Tubulin microtubules are organized as train tracks between the cortical and the endoplasm in the vegetal pole. The result is the appearance of a gray area, the gray crescent, which marks the future dorsal side of the embryo. Between the midpoint of the gray crescent and the entry point of sperm is the level of the lateral symmetry normally marked with the first cleavage.
The gray crescent is a region of zygote in which early morphogenetic movements will begin to form the blastopore, which marks the beginning of the process of gastrulation in the early embryo. Following fertilization, a sequence of cell divisions results in the formation of the early embryo. The zygote begins to divide mitotically (cleavage), and the resulting cells are termed blastomeres. As long as cleavage progresses, the cells proliferate and become progressively smaller, and the fetal size and general shape remains the same ( 73 ).
The first cleavage begins as 'a ring' (cleavage furrow) around the zygote and is parallel to the polar axis (animal-vegetal pole) of the zygote and normal to the longitudinal axis (axis joining the poles of the spindle) of the mitotic spindle. The ring consists of actin-myosin found in the cortical separating the zygote inwardly. The myosin plays the role of ATPase and controls the actin. The level of the second cleavage is parallel to the polar axis. At this stage, the embryo consists of four blastomeres, extending from the animal to the vegetal pole. Cleavage is a fractionating process, resulting in the formation of an eight-cell stage and in a 16–32 cell so-called morula ( 69 ).
5. Fertilization failure
Some fertility issues may arise from failure in fertilization events, in the molecular base, described in this review. Defects in the expression of receptors in the oocyte cell membrane (e.g., CD9), destruction of the gamete fusion mechanism, insufficient or abnormal variation of Ca 2+ and failure of exocytosis of cortical granules, inadequate completion of second meiotic division of the oocyte, defective sperm chromatin decondensation, defective pronucleus due to abnormal remodeling of chromatin (multiple pronuclei) or abnormal formation/function of the mitotic spindle, may account for infertility.
Thank you for visiting nature.com. You are using a browser version with limited support for CSS. To obtain the best experience, we recommend you use a more up to date browser (or turn off compatibility mode in Internet Explorer). In the meantime, to ensure continued support, we are displaying the site without styles and JavaScript.
- View all journals
- Explore content
- About the journal
- Publish with us
- Sign up for alerts
- Review Article
- Published: 16 November 2020
Stem-cell-based embryo models for fundamental research and translation
- Jianping Fu ORCID: orcid.org/0000-0001-9629-6739 1 , 2 , 3 ,
- Aryeh Warmflash ORCID: orcid.org/0000-0002-5679-2268 4 , 5 &
- Matthias P. Lutolf ORCID: orcid.org/0000-0002-5898-305X 6 , 7
Nature Materials volume 20 , pages 132–144 ( 2021 ) Cite this article
15k Accesses
91 Citations
42 Altmetric
Metrics details
- Biomedical engineering
- Developmental biology
- Embryonic stem cells
Despite its importance, understanding the early phases of human development has been limited by availability of human samples. The recent emergence of stem-cell-derived embryo models, a new field aiming to use stem cells to construct in vitro models to recapitulate snapshots of the development of the mammalian conceptus, opens up exciting opportunities to promote fundamental understanding of human development and advance reproductive and regenerative medicine. This Review provides a summary of the current knowledge of early mammalian development, using mouse and human conceptuses as models, and emphasizes their similarities and critical differences. We then highlight existing embryo models that mimic different aspects of mouse and human development. We further discuss bioengineering tools used for controlling multicellular interactions and self-organization critical for the development of these models. We conclude with a discussion of the important next steps and exciting future opportunities of stem-cell-derived embryo models for fundamental discovery and translation.
This is a preview of subscription content, access via your institution
Access options
Access Nature and 54 other Nature Portfolio journals
Get Nature+, our best-value online-access subscription
24,99 € / 30 days
cancel any time
Subscribe to this journal
Receive 12 print issues and online access
251,40 € per year
only 20,95 € per issue
Buy this article
- Purchase on SpringerLink
- Instant access to full article PDF
Prices may be subject to local taxes which are calculated during checkout
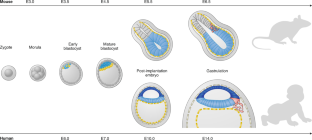
A. Yoney/E. D. Siggia ( a ); N. Rivron ( b ); Y. Zheng ( c ); M. Simunovic ( d )
Similar content being viewed by others
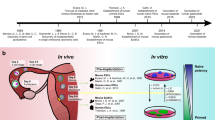
A new era of stem cell and developmental biology: from blastoids to synthetic embryos and beyond
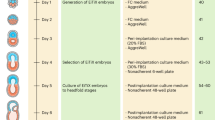
Assembly of complete mouse embryo models from embryonic and induced stem cell types in vitro
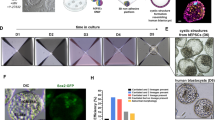
Reconstructing aspects of human embryogenesis with pluripotent stem cells
Solnica-Krezel, L. (ed.) Gastrulation: From Embryonic Pattern to Form (Academic Press, 2020).
Rossant, J. & Tam, P. P. L. New insights into early human development: lessons for stem cell derivation and differentiation. Cell Stem Cell 20 , 18–28 (2017).
CAS Google Scholar
Fogarty, N. M. E. et al. Genome editing reveals a role for OCT4 in human embryogenesis. Nature 550 , 67–73 (2017).
Ma, H. et al. Correction of a pathogenic gene mutation in human embryos. Nature 548 , 413–419 (2017).
Deglincerti, A. et al. Self-organization of the in vitro attached human embryo. Nature 533 , 251–254 (2016).
Shahbazi, M. N. et al. Self-organization of the human embryo in the absence of maternal tissues. Nat. Cell Biol. 18 , 700–708 (2016).
Xiang, L. et al. A developmental landscape of 3D-cultured human pre-gastrulation embryos. Nature 577 , 537–542 (2020).
Daley, G. Q. et al. Setting global standards for stem cell research and clinical translation: the 2016 ISSCR guidelines. Stem Cell Rep. 6 , 787–797 (2016).
Google Scholar
Hyun, I., Wilkerson, A. & Johnston, J. Revisit the 14-day rule. Nature 533 , 169–171 (2016).
Ma, H. et al. In vitro culture of cynomolgus monkey embryos beyond early gastrulation. Science 366 , eaax7890 (2019).
Niu, Y. et al. Dissecting primate early post-implantation development using long-term in vitro embryo culture. Science 366 , eaaw5754 (2019).
Nakamura, T. et al. A developmental coordinate of pluripotency among mice, monkeys and humans. Nature 537 , 57–62 (2016).
ten Berge, D. et al. Wnt signaling mediates self-organization and axis formation in embryoid bodies. Cell Stem Cell 3 , 508–518 (2008).
Fuchs, C. et al. Self-organization phenomena in embryonic stem cell-derived embryoid bodies: axis formation and breaking of symmetry during cardiomyogenesis. Cells Tissues Organs 195 , 377–391 (2012).
Meinhardt, A. et al. 3D reconstitution of the patterned neural tube from embryonic stem cells. Stem Cell Rep. 3 , 987–999 (2014).
Poh, Y.-C. et al. Generation of organized germ layers from a single mouse embryonic stem cell. Nat. Commun. 5 , 4000 (2014).
van den Brink, S. C. et al. Symmetry breaking, germ layer specification and axial organisation in aggregates of mouse embryonic stem cells. Development 141 , 4231–4242 (2014).
Warmflash, A. et al. A method to recapitulate early embryonic spatial patterning in human embryonic stem cells. Nat. Methods 11 , 847–854 (2014).
Harrison, S. E. et al. Assembly of embryonic and extra-embryonic stem cells to mimic embryogenesis in vitro. Science 356 , eaal1810 (2017).
Shao, Y. et al. A pluripotent stem cell-based model for post-implantation human amniotic sac development. Nat. Commun. 8 , 208 (2017).
Beccari, L. et al. Multi-axial self-organization properties of mouse embryonic stem cells into gastruloids. Nature 562 , 272–276 (2018).
Rivron, N. C. et al. Blastocyst-like structures generated solely from stem cells. Nature 557 , 106–111 (2018).
Sozen, B. et al. Self-assembly of embryonic and two extra-embryonic stem cell types into gastrulating embryo-like structures. Nat. Cell Biol. 20 , 979–989 (2018).
Xue, X. et al. Mechanics-guided embryonic patterning of neuroectoderm tissue from human pluripotent stem cells. Nat. Mater. 17 , 633–641 (2018).
Haremaki, T. et al. Self-organizing neuruloids model developmental aspects of Huntington’s disease in the ectodermal compartment. Nat. Biotechnol. 37 , 1198–1208 (2019).
Simunovic, M. et al. A 3D model of a human epiblast reveals BMP4-driven symmetry breaking. Nat. Cell Biol. 21 , 900–910 (2019).
Zheng, Y. et al. Controlled modelling of human epiblast and amnion development using stem cells. Nature 573 , 421–425 (2019).
van den Brink, S. C. et al. Single-cell and spatial transcriptomics reveal somitogenesis in gastruloids. Nature 582 , 405–409 (2020).
Zheng, Y. et al. Dorsal-ventral patterned neural cyst from human pluripotent stem cells in a neurogenic niche. Sci. Adv. 5 , eaax5933 (2019).
Li, R. et al. Generation of blastocyst-like structures from mouse embryonic and adult cell cultures. Cell 179 , 687–702 (2019).
Rossant, J. & Tam, P. P. L. Blastocyst lineage formation, early embryonic asymmetries and axis patterning in the mouse. Development 136 , 701–713 (2009).
Wennekamp, S., Mesecke, S., Nédélec, F. & Hiiragi, T. A self-organization framework for symmetry breaking in the mammalian embryo. Nat. Rev. Mol. Cell Biol. 14 , 452–459 (2013).
Boroviak, T. & Nichols, J. Primate embryogenesis predicts the hallmarks of human naïve pluripotency. Development 144 , 175–186 (2017).
O’Rahilly, R. & Müller, F. Developmental Stages in Human Embryos: Revised and New Measurements. Cells Tissues Organs 192 , 73–84 (2010).
Tam, P. P. L. & Loebel, D. A. F. Gene function in mouse embryogenesis: get set for gastrulation. Nat. Rev. Genet. 8 , 368–381 (2007).
Arnold, S. J. & Robertson, E. J. Making a commitment: cell lineage allocation and axis patterning in the early mouse embryo. Nat. Rev. Mol. Cell Biol. 10 , 91–103 (2009).
Rivera-Pérez, J. A., Mager, J. & Magnuson, T. Dynamic morphogenetic events characterize the mouse visceral endoderm. Dev. Biol. 261 , 470–487 (2003).
Thomas, P. & Beddington, R. Anterior primitive endoderm may be responsible for patterning the anterior neural plate in the mouse embryo. Curr. Biol. 6 , 1487–1496 (1996).
Yamamoto, M. et al. Nodal antagonists regulate formation of the anteroposterior axis of the mouse embryo. Nature 428 , 387–392 (2004).
Yoon, Y. et al. Extra-embryonic Wnt3 regulates the establishment of the primitive streak in mice. Dev. Biol. 403 , 80–88 (2015).
Tortelote, G. G. et al. Wnt3 function in the epiblast is required for the maintenance but not the initiation of gastrulation in mice. Dev. Biol. 374 , 164–173 (2013).
Ohinata, Y. et al. Blimp1 is a critical determinant of the germ cell lineage in mice. Nature 436 , 207–213 (2005).
Saitou, M., Barton, S. C. & Surani, M. A. A molecular programme for the specification of germ cell fate in mice. Nature 418 , 293–300 (2002).
Chen, D. et al. Human primordial germ cells are specified from lineage-primed progenitors. Cell Rep. 29 , 4568–4582e4565 (2019).
Sasaki, K. et al. The germ cell fate of cynomolgus monkeys is specified in the nascent amnion. Dev. Cell 39 , 169–185 (2016).
Colas, J.-F. & Schoenwolf, G. C. Towards a cellular and molecular understanding of neurulation. Dev. Dynam. 221 , 117–145 (2001).
Vijayraghavan, D. S. & Davidson, L. A. Mechanics of neurulation: from classical to current perspectives on the physical mechanics that shape, fold, and form the neural tube. Birth Defects Res. 109 , 153–168 (2017).
Ribes, V. & Briscoe, J. Establishing and interpreting graded sonic hedgehog signaling during vertebrate neural tube patterning: The role of negative feedback. Cold Spring Harbor Persp. Biol. 1 , a002014 (2009).
Briscoe, J. & Small, S. Morphogen rules: Design principles of gradient-mediated embryo patterning. Development 142 , 3996–4009 (2015).
Nichols, J. & Smith, A. Naive and primed pluripotent states. Cell Stem Cell 4 , 487–492 (2009).
Smith, A. Formative pluripotency: the executive phase in a developmental continuum. Development 144 , 365–373 (2017).
Boroviak, T. et al. The ability of inner-cell-mass cells to self-renew as embryonic stem cells is acquired following epiblast specification. Nat. Cell Biol. 16 , 513–525 (2014).
Ying, Q.-L. et al. The ground state of embryonic stem cell self-renewal. Nature 453 , 519–523 (2008).
Brons, I. G. M. et al. Derivation of pluripotent epiblast stem cells from mammalian embryos. Nature 448 , 191–195 (2007).
Tesar, P. J. et al. New cell lines from mouse epiblast share defining features with human embryonic stem cells. Nature 448 , 196–199 (2007).
Kojima, Y. et al. The transcriptional and functional properties of mouse epiblast stem cells resemble the anterior primitive streak. Cell Stem Cell 14 , 107–120 (2014).
Hayashi, K. et al. Reconstitution of the mouse germ cell specification pathway in culture by pluripotent stem cells. Cell 146 , 519–532 (2011).
Tanaka, S. et al. Promotion of trophoblast stem cell proliferation by FGF4. Science 282 , 2072–2075 (1998).
Niakan, K. K., Schrode, N., Cho, L. T. Y. & Hadjantonakis, A.-K. Derivation of extraembryonic endoderm stem (XEN) cells from mouse embryos and embryonic stem cells. Nat. Protocols 8 , 1028–1041 (2013).
Thomson, J. A. et al. Embryonic stem cell lines derived from human blastocysts. Science 282 , 1145–1147 (1998).
Guo, H. et al. The DNA methylation landscape of human early embryos. Nature 511 , 606–610 (2014).
Yan, L. et al. Single-cell RNA-Seq profiling of human preimplantation embryos and embryonic stem cells. Nature Struct. Mol. Biol. 20 , 1131–1139 (2013).
Chia, N.-Y. et al. A genome-wide RNAi screen reveals determinants of human embryonic stem cell identity. Nature 468 , 316–320 (2010).
Irie, N. et al. SOX17 is a critical specifier of human primordial germ cell fate. Cell 160 , 253–268 (2015).
Sasaki, K. et al. Robust in vitro induction of human germ cell fate from pluripotent stem cells. Cell Stem Cell 17 , 178–194 (2015).
Theunissen, T. W. et al. Systematic identification of culture conditions for induction and maintenance of naive human pluripotency. Cell Stem Cell 15 , 471–487 (2014).
Guo, G. et al. Naive pluripotent stem cells derived directly from isolated cells of the human inner cell mass. Stem Cell Rep. 6 , 437–446 (2016).
Theunissen, T. W. et al. Molecular criteria for defining the naive human pluripotent state. Cell Stem Cell 19 , 502–515 (2016).
Takashima, Y. et al. Resetting transcription factor control circuitry toward ground-state pluripotency in human. Cell 158 , 1254–1269 (2014).
Yamanaka, S. & Blau, H. M. Nuclear reprogramming to a pluripotent state by three approaches. Nature 465 , 704–712 (2010).
Choi, J. et al. A comparison of genetically matched cell lines reveals the equivalence of human iPSCs and ESCs. Nat. Biotechnol. 33 , 1173–1181 (2015).
Quadrato, G. et al. Cell diversity and network dynamics in photosensitive human brain organoids. Nature 545 , 48–53 (2017).
Yang, Y. et al. Derivation of pluripotent stem cells with in vivo embryonic and extraembryonic potency. Cell 169 , 243–257 (2017).
Gao, X. et al. Establishment of porcine and human expanded potential stem cells. Nat. Cell Biol. 21 , 687–699 (2019).
Okae, H. et al. Derivation of human trophoblast stem cells. Cell Stem Cell 22 , 50–63e56 (2018).
Linneberg-Agerholm, M. et al. Naïve human pluripotent stem cells respond to Wnt, Nodal and LIF signalling to produce expandable naïve extra-embryonic endoderm. Development 146 , dev180620 (2019).
Dong, C. et al. Derivation of trophoblast stem cells from naïve human pluripotent stem cells. eLife 9 , e52504 (2019).
Guo, G. et al. Trophectoderm potency is retained exclusively in human naïve cells. Preprint at https://doi.org/10.1101/2020.02.04.933812 (2020).
Posfai, E. et al. Defining totipotency using criteria of increasing stringency. Preprint at https://doi.org/10.1101/2020.03.02.972893 (2020).
Frias-Aldeguer, J. et al. Embryonic signals perpetuate polar-like trophoblast stem cells and pattern the blastocyst axis. Preprint at https://doi.org/10.1101/510362 (2019).
Vrij, E. J. et al. Chemically-defined induction of a primitive endoderm and epiblast-like niche supports post-implantation progression from blastoids. Preprint at https://doi.org/10.1101/510396 (2019).
Sozen, B. et al. Self-organization of mouse stem cells into an extended potential blastoid. Dev. Cell 51 , 698–712 (2019).
Taniguchi, K. et al. Lumen formation is an intrinsic property of isolated human pluripotent stem cells. Stem Cell Rep. 5 , 954–962 (2015).
Shahbazi, M. N. et al. Pluripotent state transitions coordinate morphogenesis in mouse and human embryos. Nature 552 , 239–243 (2017).
Minn, K. T. et al. High-resolution transcriptional and morphogenetic profiling of cells from micropatterned human embryonic stem cell gastruloid cultures. Preprint at https://doi.org/10.1101/2020.01.22.915777 (2020).
Tewary, M. et al. A stepwise model of reaction-diffusion and positional-information governs self-organized human peri-gastrulation-like patterning. Development 144 , 4298–4312 (2017).
Etoc, F. et al. A balance between secreted inhibitors and edge sensing controls gastruloid self-organization. Dev. Cell 39 , 302–315 (2016).
Heemskerk, I. et al. Rapid changes in morphogen concentration control self-organized patterning in human embryonic stem cells. eLife 8 , e40526 (2019).
Nemashkalo, A., Ruzo, A., Heemskerk, I. & Warmflash, A. Morphogen and community effects determine cell fates in response to BMP4 signaling in human embryonic stem cells. Development 144 , 3042–3053 (2017).
Chhabra, S. et al. Dissecting the dynamics of signaling events in the BMP, WNT, and NODAL cascade during self-organized fate patterning in human gastruloids. PLoS Biol. 17 , e3000498 (2019).
Martyn, I., Brivanlou, A. H. & Siggia, E. D. A wave of WNT signaling balanced by secreted inhibitors controls primitive streak formation in micropattern colonies of human embryonic stem cells. Development 146 , dev172791 (2019).
Morgani, S. M. et al. Micropattern differentiation of mouse pluripotent stem cells recapitulates embryo regionalized cell fate patterning. eLife 7 , e32839 (2018).
Manfrin, A. et al. Engineered signaling centers for the spatially controlled patterning of human pluripotent stem cells. Nat. Methods 16 , 640–648 (2019).
Veenvliet, J. V. et al. Mouse embryonic stem cells self-organize into trunk-like structures with neural tube and somites. Preprint at https://doi.org/10.1101/2020.03.04.974949 (2020).
Rossi, G. et al. Embryonic organoids recapitulate early heart organogenesis. Preprint at https://doi.org/10.1101/802181 (2019).
Ranga, A. et al. Neural tube morphogenesis in synthetic 3D microenvironments. Proc. Natl Acad. Sci. USA 113 , E6831–E6839 (2016).
Britton, G. et al. A novel self-organizing embryonic stem cell system reveals signaling logic underlying the patterning of human ectoderm. Development 146 , dev179093 (2019).
McBeath, R. et al. Cell shape, cytoskeletal tension, and RhoA regulate stem cell lineage commitment. Dev. Cell 6 , 483–495 (2004).
Lam, R. H. W., Sun, Y., Chen, W. & Fu, J. Elastomeric microposts integrated into microfluidics for flow-mediated endothelial mechanotransduction analysis. Lab Chip 12 , 1865–1873 (2012).
Nelson, C. M. et al. Tissue geometry determines sites of mammary branching morphogenesis in organotypic cultures. Science 314 , 298–300 (2006).
Habib, S. J. et al. A localized Wnt signal orients asymmetric stem cell division in vitro. Science 339 , 1445–1448 (2013).
Repina, N. A. et al. Optogenetic control of Wnt signaling for modeling early embryogenic patterning with human pluripotent stem cells. Preprint at https://doi.org/10.1101/665695 (2019).
Cederquist, G. Y. et al. Specification of positional identity in forebrain organoids. Nat. Biotechnol. 37 , 436–444 (2019).
Sun, Y., Chen, C. S. & Fu, J. Forcing stem cells to behave: A biophysical perspective of the cellular microenvironment. Annu. Rev. Biophys. 41 , 519–542 (2012).
Bedzhov, I. & Zernicka-Goetz, M. Self-organizing properties of mouse pluripotent cells initiate morphogenesis upon implantation. Cell 156 , 1032–1044 (2014).
Tam, P. P. Postimplantation mouse development: whole embryo culture and micro-manipulation. Int. J. Dev. Biol. 42 , 895–902 (1998).
Wang, H. et al. A novel model of human implantation: 3D endometrium-like culture system to study attachment of human trophoblast (Jar) cell spheroids. Mol. Human Reprod. 18 , 33–43 (2011).
Tyser, R. C. V. et al. A spatially resolved single cell atlas of human gastrulation. Preprint at https://doi.org/10.1101/2020.07.21.213512 (2020).
Rivron, N. et al. Debate ethics of embryo models from stem cells. Nature 564 , 183–185 (2018).
Hyun, I. et al. Toward guidelines for research on human embryo models formed from stem cells. Stem Cell Rep. 14 , 169–174 (2020).
Petropoulos, S. et al. Single-Cell RNA-Seq reveals lineage and X chromosome dynamics in human preimplantation embryos. Cell 165 , 1012–1026 (2016).
Dobreva, M. P. et al. Amniotic ectoderm expansion in mouse occurs via distinct modes and requires SMAD5-mediated signalling. Development 145 , dev157222 (2018).
Pereira, P. N. G. et al. Amnion formation in the mouse embryo: the single amniochorionic fold model. BMC Dev. Biol. 11 , 48 (2011).
Lawson, K. A. et al. Bmp4 is required for the generation of primordial germ cells in the mouse embryo. Genes Dev. 13 , 424–436 (1999).
Munoz-Sanjuan, I. & Brivanlou, A. H. Neural induction, the default model and embryonic stem cells. Nat. Rev. Neurosci. 3 , 271–280 (2002).
Stern, C. D. Neural induction: old problem, new findings, yet more questions. Development 132 , 2007–2021 (2005).
Barnat, M. et al. Huntington’s disease alters human neurodevelopment. Science 369 , 787–793 (2020).
Download references
Acknowledgements
The authors thank S. Vianello for invaluable work on illustrations and N. Rivron, A. Yoney, E.D. Siggia, M. Simunovic and Y. Zheng for microscopic images shown in Fig. 4 . J.F.’s research is supported by the University of Michigan Mechanical Engineering Department, the Michigan–Cambridge Research Initiative, the University of Michigan Mcubed Fund, the National Institutes of Health (R21 NS113518 and R21 HD100931), and the National Science Foundation (CMMI 1917304 and CBET 1901718). A.W.’s work is supported by the Rice University, the Welch Foundation (C-2021), the Simons Foundation (511709), the National Institutes of Health (R01 GM126122), and the National Science Foundation (MCB-1553228). M.P.L.’s work is supported by the École Polytechnique Fédérale de Lausanne, the National Center of Competence in Research (NCCR) ‘Bio-Inspired Materials’, and the Swiss National Science Foundation Sinergia Grant (no. 3189956). The authors apologize to colleagues whose work they could not cite owing to space restrictions.
Author information
Authors and affiliations.
Department of Mechanical Engineering, University of Michigan, Ann Arbor, MI, USA
Jianping Fu
Department of Biomedical Engineering, University of Michigan, Ann Arbor, MI, USA
Department of Cell and Developmental Biology, University of Michigan Medical School, Ann Arbor, MI, USA
Department of Biosciences, Rice University, Houston, TX, USA
- Aryeh Warmflash
Department of Bioengineering, Rice University, Houston, TX, USA
Laboratory of Stem Cell Bioengineering, Institute of Bioengineering, School of Life Sciences and School of Engineering, École Polytechnique Fédérale de Lausanne, Lausanne, Switzerland
Matthias P. Lutolf
Institute of Chemical Sciences and Engineering, School of Basic Science, École Polytechnique Fédérale de Lausanne, Lausanne, Switzerland
You can also search for this author in PubMed Google Scholar
Contributions
J.F., A.W. and M.P.L. wrote the manuscript. All authors edited and approved the manuscript.
Corresponding authors
Correspondence to Jianping Fu , Aryeh Warmflash or Matthias P. Lutolf .
Ethics declarations
Competing interests.
The authors declare no competing interests.
Additional information
Publisher’s note Springer Nature remains neutral with regard to jurisdictional claims in published maps and institutional affiliations.
Rights and permissions
Reprints and permissions
About this article
Cite this article.
Fu, J., Warmflash, A. & Lutolf, M.P. Stem-cell-based embryo models for fundamental research and translation. Nat. Mater. 20 , 132–144 (2021). https://doi.org/10.1038/s41563-020-00829-9
Download citation
Received : 14 April 2020
Accepted : 14 September 2020
Published : 16 November 2020
Issue Date : February 2021
DOI : https://doi.org/10.1038/s41563-020-00829-9
Share this article
Anyone you share the following link with will be able to read this content:
Sorry, a shareable link is not currently available for this article.
Provided by the Springer Nature SharedIt content-sharing initiative
This article is cited by
Precise and scalable self-organization in mammalian pseudo-embryos.
- Mélody Merle
- Leah Friedman
- Thomas Gregor
Nature Structural & Molecular Biology (2024)
Time-integrated BMP signaling determines fate in a stem cell model for early human development
- Seth Teague
- Gillian Primavera
- Idse Heemskerk
Nature Communications (2024)
Reprogramming fibroblast into human iBlastoids
- Jia Ping Tan
- Xiaodong Liu
- Jose M. Polo
Nature Protocols (2024)
Criteria for the standardization of stem-cell-based embryo models
- Alfonso Martinez Arias
- Nicolas Rivron
Nature Cell Biology (2024)
Substrates mimicking the blastocyst geometry revert pluripotent stem cell to naivety
- Weiwei Wang
Nature Materials (2024)
Quick links
- Explore articles by subject
- Guide to authors
- Editorial policies
Sign up for the Nature Briefing newsletter — what matters in science, free to your inbox daily.

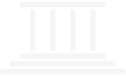
Subject Area and Category
- Cell Biology
- Developmental Biology
Cambridge University Press
Publication type
09671994, 14698730
Information
How to publish in this journal
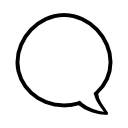
The set of journals have been ranked according to their SJR and divided into four equal groups, four quartiles. Q1 (green) comprises the quarter of the journals with the highest values, Q2 (yellow) the second highest values, Q3 (orange) the third highest values and Q4 (red) the lowest values.
Category | Year | Quartile |
---|---|---|
Cell Biology | 1999 | Q4 |
Cell Biology | 2000 | Q4 |
Cell Biology | 2001 | Q4 |
Cell Biology | 2002 | Q3 |
Cell Biology | 2003 | Q4 |
Cell Biology | 2004 | Q3 |
Cell Biology | 2005 | Q3 |
Cell Biology | 2006 | Q4 |
Cell Biology | 2007 | Q3 |
Cell Biology | 2008 | Q4 |
Cell Biology | 2009 | Q4 |
Cell Biology | 2010 | Q4 |
Cell Biology | 2011 | Q4 |
Cell Biology | 2012 | Q4 |
Cell Biology | 2013 | Q4 |
Cell Biology | 2014 | Q4 |
Cell Biology | 2015 | Q4 |
Cell Biology | 2016 | Q4 |
Cell Biology | 2017 | Q4 |
Cell Biology | 2018 | Q4 |
Cell Biology | 2019 | Q4 |
Cell Biology | 2020 | Q4 |
Cell Biology | 2021 | Q4 |
Cell Biology | 2022 | Q4 |
Cell Biology | 2023 | Q4 |
Developmental Biology | 1999 | Q4 |
Developmental Biology | 2000 | Q4 |
Developmental Biology | 2001 | Q4 |
Developmental Biology | 2002 | Q3 |
Developmental Biology | 2003 | Q4 |
Developmental Biology | 2004 | Q3 |
Developmental Biology | 2005 | Q3 |
Developmental Biology | 2006 | Q4 |
Developmental Biology | 2007 | Q3 |
Developmental Biology | 2008 | Q3 |
Developmental Biology | 2009 | Q4 |
Developmental Biology | 2010 | Q4 |
Developmental Biology | 2011 | Q4 |
Developmental Biology | 2012 | Q4 |
Developmental Biology | 2013 | Q4 |
Developmental Biology | 2014 | Q4 |
Developmental Biology | 2015 | Q4 |
Developmental Biology | 2016 | Q4 |
Developmental Biology | 2017 | Q4 |
Developmental Biology | 2018 | Q4 |
Developmental Biology | 2019 | Q4 |
Developmental Biology | 2020 | Q4 |
Developmental Biology | 2021 | Q4 |
Developmental Biology | 2022 | Q4 |
Developmental Biology | 2023 | Q4 |
The SJR is a size-independent prestige indicator that ranks journals by their 'average prestige per article'. It is based on the idea that 'all citations are not created equal'. SJR is a measure of scientific influence of journals that accounts for both the number of citations received by a journal and the importance or prestige of the journals where such citations come from It measures the scientific influence of the average article in a journal, it expresses how central to the global scientific discussion an average article of the journal is.
Year | SJR |
---|---|
1999 | 0.368 |
2000 | 0.356 |
2001 | 0.298 |
2002 | 0.445 |
2003 | 0.435 |
2004 | 0.562 |
2005 | 0.545 |
2006 | 0.437 |
2007 | 0.593 |
2008 | 0.527 |
2009 | 0.508 |
2010 | 0.576 |
2011 | 0.505 |
2012 | 0.468 |
2013 | 0.539 |
2014 | 0.427 |
2015 | 0.391 |
2016 | 0.376 |
2017 | 0.387 |
2018 | 0.470 |
2019 | 0.435 |
2020 | 0.416 |
2021 | 0.363 |
2022 | 0.369 |
2023 | 0.414 |
Evolution of the number of published documents. All types of documents are considered, including citable and non citable documents.
Year | Documents |
---|---|
1999 | 43 |
2000 | 95 |
2001 | 41 |
2002 | 45 |
2003 | 44 |
2004 | 45 |
2005 | 42 |
2006 | 37 |
2007 | 38 |
2008 | 42 |
2009 | 40 |
2010 | 41 |
2011 | 42 |
2012 | 52 |
2013 | 51 |
2014 | 114 |
2015 | 58 |
2016 | 98 |
2017 | 78 |
2018 | 59 |
2019 | 80 |
2020 | 82 |
2021 | 76 |
2022 | 86 |
2023 | 90 |
This indicator counts the number of citations received by documents from a journal and divides them by the total number of documents published in that journal. The chart shows the evolution of the average number of times documents published in a journal in the past two, three and four years have been cited in the current year. The two years line is equivalent to journal impact factor ™ (Thomson Reuters) metric.
Cites per document | Year | Value |
---|---|---|
Cites / Doc. (4 years) | 1999 | 0.632 |
Cites / Doc. (4 years) | 2000 | 0.901 |
Cites / Doc. (4 years) | 2001 | 0.681 |
Cites / Doc. (4 years) | 2002 | 0.903 |
Cites / Doc. (4 years) | 2003 | 1.254 |
Cites / Doc. (4 years) | 2004 | 1.218 |
Cites / Doc. (4 years) | 2005 | 1.383 |
Cites / Doc. (4 years) | 2006 | 1.119 |
Cites / Doc. (4 years) | 2007 | 1.571 |
Cites / Doc. (4 years) | 2008 | 1.407 |
Cites / Doc. (4 years) | 2009 | 1.208 |
Cites / Doc. (4 years) | 2010 | 1.420 |
Cites / Doc. (4 years) | 2011 | 1.441 |
Cites / Doc. (4 years) | 2012 | 1.297 |
Cites / Doc. (4 years) | 2013 | 1.240 |
Cites / Doc. (4 years) | 2014 | 1.263 |
Cites / Doc. (4 years) | 2015 | 0.907 |
Cites / Doc. (4 years) | 2016 | 1.080 |
Cites / Doc. (4 years) | 2017 | 1.153 |
Cites / Doc. (4 years) | 2018 | 1.172 |
Cites / Doc. (4 years) | 2019 | 1.259 |
Cites / Doc. (4 years) | 2020 | 1.508 |
Cites / Doc. (4 years) | 2021 | 1.756 |
Cites / Doc. (4 years) | 2022 | 1.549 |
Cites / Doc. (4 years) | 2023 | 1.522 |
Cites / Doc. (3 years) | 1999 | 0.632 |
Cites / Doc. (3 years) | 2000 | 0.829 |
Cites / Doc. (3 years) | 2001 | 0.581 |
Cites / Doc. (3 years) | 2002 | 1.201 |
Cites / Doc. (3 years) | 2003 | 1.171 |
Cites / Doc. (3 years) | 2004 | 1.315 |
Cites / Doc. (3 years) | 2005 | 1.291 |
Cites / Doc. (3 years) | 2006 | 1.092 |
Cites / Doc. (3 years) | 2007 | 1.500 |
Cites / Doc. (3 years) | 2008 | 1.376 |
Cites / Doc. (3 years) | 2009 | 1.308 |
Cites / Doc. (3 years) | 2010 | 1.408 |
Cites / Doc. (3 years) | 2011 | 1.350 |
Cites / Doc. (3 years) | 2012 | 1.276 |
Cites / Doc. (3 years) | 2013 | 1.267 |
Cites / Doc. (3 years) | 2014 | 1.124 |
Cites / Doc. (3 years) | 2015 | 0.903 |
Cites / Doc. (3 years) | 2016 | 1.040 |
Cites / Doc. (3 years) | 2017 | 1.148 |
Cites / Doc. (3 years) | 2018 | 1.209 |
Cites / Doc. (3 years) | 2019 | 1.345 |
Cites / Doc. (3 years) | 2020 | 1.447 |
Cites / Doc. (3 years) | 2021 | 1.597 |
Cites / Doc. (3 years) | 2022 | 1.563 |
Cites / Doc. (3 years) | 2023 | 1.529 |
Cites / Doc. (2 years) | 1999 | 0.482 |
Cites / Doc. (2 years) | 2000 | 0.660 |
Cites / Doc. (2 years) | 2001 | 0.797 |
Cites / Doc. (2 years) | 2002 | 1.037 |
Cites / Doc. (2 years) | 2003 | 1.372 |
Cites / Doc. (2 years) | 2004 | 1.202 |
Cites / Doc. (2 years) | 2005 | 1.146 |
Cites / Doc. (2 years) | 2006 | 0.793 |
Cites / Doc. (2 years) | 2007 | 1.532 |
Cites / Doc. (2 years) | 2008 | 1.213 |
Cites / Doc. (2 years) | 2009 | 1.263 |
Cites / Doc. (2 years) | 2010 | 1.268 |
Cites / Doc. (2 years) | 2011 | 1.111 |
Cites / Doc. (2 years) | 2012 | 1.349 |
Cites / Doc. (2 years) | 2013 | 0.979 |
Cites / Doc. (2 years) | 2014 | 1.039 |
Cites / Doc. (2 years) | 2015 | 0.830 |
Cites / Doc. (2 years) | 2016 | 0.930 |
Cites / Doc. (2 years) | 2017 | 1.160 |
Cites / Doc. (2 years) | 2018 | 1.313 |
Cites / Doc. (2 years) | 2019 | 1.292 |
Cites / Doc. (2 years) | 2020 | 1.144 |
Cites / Doc. (2 years) | 2021 | 1.488 |
Cites / Doc. (2 years) | 2022 | 1.411 |
Cites / Doc. (2 years) | 2023 | 1.531 |
Evolution of the total number of citations and journal's self-citations received by a journal's published documents during the three previous years. Journal Self-citation is defined as the number of citation from a journal citing article to articles published by the same journal.
Cites | Year | Value |
---|---|---|
Self Cites | 1999 | 18 |
Self Cites | 2000 | 17 |
Self Cites | 2001 | 10 |
Self Cites | 2002 | 18 |
Self Cites | 2003 | 19 |
Self Cites | 2004 | 16 |
Self Cites | 2005 | 12 |
Self Cites | 2006 | 13 |
Self Cites | 2007 | 11 |
Self Cites | 2008 | 23 |
Self Cites | 2009 | 18 |
Self Cites | 2010 | 14 |
Self Cites | 2011 | 8 |
Self Cites | 2012 | 8 |
Self Cites | 2013 | 16 |
Self Cites | 2014 | 26 |
Self Cites | 2015 | 20 |
Self Cites | 2016 | 33 |
Self Cites | 2017 | 24 |
Self Cites | 2018 | 18 |
Self Cites | 2019 | 19 |
Self Cites | 2020 | 20 |
Self Cites | 2021 | 17 |
Self Cites | 2022 | 6 |
Self Cites | 2023 | 23 |
Total Cites | 1999 | 151 |
Total Cites | 2000 | 194 |
Total Cites | 2001 | 168 |
Total Cites | 2002 | 215 |
Total Cites | 2003 | 212 |
Total Cites | 2004 | 171 |
Total Cites | 2005 | 173 |
Total Cites | 2006 | 143 |
Total Cites | 2007 | 186 |
Total Cites | 2008 | 161 |
Total Cites | 2009 | 153 |
Total Cites | 2010 | 169 |
Total Cites | 2011 | 166 |
Total Cites | 2012 | 157 |
Total Cites | 2013 | 171 |
Total Cites | 2014 | 163 |
Total Cites | 2015 | 196 |
Total Cites | 2016 | 232 |
Total Cites | 2017 | 310 |
Total Cites | 2018 | 283 |
Total Cites | 2019 | 316 |
Total Cites | 2020 | 314 |
Total Cites | 2021 | 353 |
Total Cites | 2022 | 372 |
Total Cites | 2023 | 373 |
Evolution of the number of total citation per document and external citation per document (i.e. journal self-citations removed) received by a journal's published documents during the three previous years. External citations are calculated by subtracting the number of self-citations from the total number of citations received by the journal’s documents.
Cites | Year | Value |
---|---|---|
External Cites per document | 1999 | 0.556 |
External Cites per document | 2000 | 0.756 |
External Cites per document | 2001 | 0.547 |
External Cites per document | 2002 | 1.101 |
External Cites per document | 2003 | 1.066 |
External Cites per document | 2004 | 1.192 |
External Cites per document | 2005 | 1.201 |
External Cites per document | 2006 | 0.992 |
External Cites per document | 2007 | 1.411 |
External Cites per document | 2008 | 1.179 |
External Cites per document | 2009 | 1.154 |
External Cites per document | 2010 | 1.292 |
External Cites per document | 2011 | 1.285 |
External Cites per document | 2012 | 1.211 |
External Cites per document | 2013 | 1.148 |
External Cites per document | 2014 | 0.945 |
External Cites per document | 2015 | 0.811 |
External Cites per document | 2016 | 0.892 |
External Cites per document | 2017 | 1.059 |
External Cites per document | 2018 | 1.132 |
External Cites per document | 2019 | 1.264 |
External Cites per document | 2020 | 1.355 |
External Cites per document | 2021 | 1.520 |
External Cites per document | 2022 | 1.538 |
External Cites per document | 2023 | 1.434 |
Cites per document | 1999 | 0.632 |
Cites per document | 2000 | 0.829 |
Cites per document | 2001 | 0.581 |
Cites per document | 2002 | 1.201 |
Cites per document | 2003 | 1.171 |
Cites per document | 2004 | 1.315 |
Cites per document | 2005 | 1.291 |
Cites per document | 2006 | 1.092 |
Cites per document | 2007 | 1.500 |
Cites per document | 2008 | 1.376 |
Cites per document | 2009 | 1.308 |
Cites per document | 2010 | 1.408 |
Cites per document | 2011 | 1.350 |
Cites per document | 2012 | 1.276 |
Cites per document | 2013 | 1.267 |
Cites per document | 2014 | 1.124 |
Cites per document | 2015 | 0.903 |
Cites per document | 2016 | 1.040 |
Cites per document | 2017 | 1.148 |
Cites per document | 2018 | 1.209 |
Cites per document | 2019 | 1.345 |
Cites per document | 2020 | 1.447 |
Cites per document | 2021 | 1.597 |
Cites per document | 2022 | 1.563 |
Cites per document | 2023 | 1.529 |
International Collaboration accounts for the articles that have been produced by researchers from several countries. The chart shows the ratio of a journal's documents signed by researchers from more than one country; that is including more than one country address.
Year | International Collaboration |
---|---|
1999 | 27.91 |
2000 | 15.79 |
2001 | 17.07 |
2002 | 26.67 |
2003 | 25.00 |
2004 | 15.56 |
2005 | 14.29 |
2006 | 18.92 |
2007 | 15.79 |
2008 | 26.19 |
2009 | 20.00 |
2010 | 19.51 |
2011 | 16.67 |
2012 | 15.38 |
2013 | 17.65 |
2014 | 21.05 |
2015 | 32.76 |
2016 | 24.49 |
2017 | 21.79 |
2018 | 30.51 |
2019 | 22.50 |
2020 | 19.51 |
2021 | 25.00 |
2022 | 27.91 |
2023 | 17.78 |
Not every article in a journal is considered primary research and therefore "citable", this chart shows the ratio of a journal's articles including substantial research (research articles, conference papers and reviews) in three year windows vs. those documents other than research articles, reviews and conference papers.
Documents | Year | Value |
---|---|---|
Non-citable documents | 1999 | 0 |
Non-citable documents | 2000 | 0 |
Non-citable documents | 2001 | 1 |
Non-citable documents | 2002 | 1 |
Non-citable documents | 2003 | 1 |
Non-citable documents | 2004 | 0 |
Non-citable documents | 2005 | 0 |
Non-citable documents | 2006 | 0 |
Non-citable documents | 2007 | 0 |
Non-citable documents | 2008 | 0 |
Non-citable documents | 2009 | 0 |
Non-citable documents | 2010 | 0 |
Non-citable documents | 2011 | 0 |
Non-citable documents | 2012 | 0 |
Non-citable documents | 2013 | 1 |
Non-citable documents | 2014 | 1 |
Non-citable documents | 2015 | 1 |
Non-citable documents | 2016 | 1 |
Non-citable documents | 2017 | 1 |
Non-citable documents | 2018 | 1 |
Non-citable documents | 2019 | 0 |
Non-citable documents | 2020 | 1 |
Non-citable documents | 2021 | 1 |
Non-citable documents | 2022 | 1 |
Non-citable documents | 2023 | 0 |
Citable documents | 1999 | 239 |
Citable documents | 2000 | 234 |
Citable documents | 2001 | 288 |
Citable documents | 2002 | 178 |
Citable documents | 2003 | 180 |
Citable documents | 2004 | 130 |
Citable documents | 2005 | 134 |
Citable documents | 2006 | 131 |
Citable documents | 2007 | 124 |
Citable documents | 2008 | 117 |
Citable documents | 2009 | 117 |
Citable documents | 2010 | 120 |
Citable documents | 2011 | 123 |
Citable documents | 2012 | 123 |
Citable documents | 2013 | 134 |
Citable documents | 2014 | 144 |
Citable documents | 2015 | 216 |
Citable documents | 2016 | 222 |
Citable documents | 2017 | 269 |
Citable documents | 2018 | 233 |
Citable documents | 2019 | 235 |
Citable documents | 2020 | 216 |
Citable documents | 2021 | 220 |
Citable documents | 2022 | 237 |
Citable documents | 2023 | 244 |
Ratio of a journal's items, grouped in three years windows, that have been cited at least once vs. those not cited during the following year.
Documents | Year | Value |
---|---|---|
Uncited documents | 1999 | 165 |
Uncited documents | 2000 | 143 |
Uncited documents | 2001 | 212 |
Uncited documents | 2002 | 83 |
Uncited documents | 2003 | 101 |
Uncited documents | 2004 | 46 |
Uncited documents | 2005 | 52 |
Uncited documents | 2006 | 55 |
Uncited documents | 2007 | 37 |
Uncited documents | 2008 | 42 |
Uncited documents | 2009 | 46 |
Uncited documents | 2010 | 49 |
Uncited documents | 2011 | 54 |
Uncited documents | 2012 | 52 |
Uncited documents | 2013 | 62 |
Uncited documents | 2014 | 61 |
Uncited documents | 2015 | 100 |
Uncited documents | 2016 | 101 |
Uncited documents | 2017 | 113 |
Uncited documents | 2018 | 102 |
Uncited documents | 2019 | 93 |
Uncited documents | 2020 | 85 |
Uncited documents | 2021 | 77 |
Uncited documents | 2022 | 87 |
Uncited documents | 2023 | 87 |
Cited documents | 1999 | 74 |
Cited documents | 2000 | 91 |
Cited documents | 2001 | 77 |
Cited documents | 2002 | 96 |
Cited documents | 2003 | 80 |
Cited documents | 2004 | 84 |
Cited documents | 2005 | 82 |
Cited documents | 2006 | 76 |
Cited documents | 2007 | 87 |
Cited documents | 2008 | 75 |
Cited documents | 2009 | 71 |
Cited documents | 2010 | 71 |
Cited documents | 2011 | 69 |
Cited documents | 2012 | 71 |
Cited documents | 2013 | 73 |
Cited documents | 2014 | 84 |
Cited documents | 2015 | 117 |
Cited documents | 2016 | 122 |
Cited documents | 2017 | 157 |
Cited documents | 2018 | 132 |
Cited documents | 2019 | 142 |
Cited documents | 2020 | 132 |
Cited documents | 2021 | 144 |
Cited documents | 2022 | 151 |
Cited documents | 2023 | 157 |
Evolution of the percentage of female authors.
Year | Female Percent |
---|---|
1999 | 34.90 |
2000 | 32.17 |
2001 | 34.07 |
2002 | 34.59 |
2003 | 34.07 |
2004 | 40.35 |
2005 | 40.12 |
2006 | 29.75 |
2007 | 34.25 |
2008 | 43.79 |
2009 | 40.80 |
2010 | 46.08 |
2011 | 38.04 |
2012 | 39.11 |
2013 | 46.48 |
2014 | 42.66 |
2015 | 45.13 |
2016 | 39.39 |
2017 | 44.92 |
2018 | 52.61 |
2019 | 46.29 |
2020 | 47.49 |
2021 | 42.03 |
2022 | 42.43 |
2023 | 46.55 |
Evolution of the number of documents cited by public policy documents according to Overton database.
Documents | Year | Value |
---|---|---|
Overton | 1999 | 0 |
Overton | 2000 | 0 |
Overton | 2001 | 0 |
Overton | 2002 | 0 |
Overton | 2003 | 0 |
Overton | 2004 | 0 |
Overton | 2005 | 0 |
Overton | 2006 | 0 |
Overton | 2007 | 0 |
Overton | 2008 | 0 |
Overton | 2009 | 0 |
Overton | 2010 | 0 |
Overton | 2011 | 0 |
Overton | 2012 | 0 |
Overton | 2013 | 0 |
Overton | 2014 | 0 |
Overton | 2015 | 0 |
Overton | 2016 | 0 |
Overton | 2017 | 0 |
Overton | 2018 | 0 |
Overton | 2019 | 0 |
Overton | 2020 | 0 |
Overton | 2021 | 0 |
Overton | 2022 | 0 |
Overton | 2023 | 0 |
Evoution of the number of documents related to Sustainable Development Goals defined by United Nations. Available from 2018 onwards.
Documents | Year | Value |
---|---|---|
SDG | 2018 | 5 |
SDG | 2019 | 11 |
SDG | 2020 | 10 |
SDG | 2021 | 13 |
SDG | 2022 | 12 |
SDG | 2023 | 12 |
Leave a comment
Name * Required
Email (will not be published) * Required
* Required Cancel
The users of Scimago Journal & Country Rank have the possibility to dialogue through comments linked to a specific journal. The purpose is to have a forum in which general doubts about the processes of publication in the journal, experiences and other issues derived from the publication of papers are resolved. For topics on particular articles, maintain the dialogue through the usual channels with your editor.
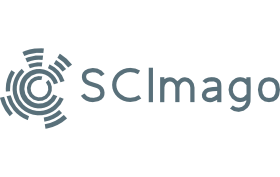
Follow us on @ScimagoJR Scimago Lab , Copyright 2007-2024. Data Source: Scopus®

Cookie settings
Cookie Policy
Legal Notice
Privacy Policy
- Open access
- Published: 26 February 2019
Stem cells: past, present, and future
- Wojciech Zakrzewski 1 ,
- Maciej Dobrzyński 2 ,
- Maria Szymonowicz 1 &
- Zbigniew Rybak 1
Stem Cell Research & Therapy volume 10 , Article number: 68 ( 2019 ) Cite this article
583k Accesses
952 Citations
54 Altmetric
Metrics details
In recent years, stem cell therapy has become a very promising and advanced scientific research topic. The development of treatment methods has evoked great expectations. This paper is a review focused on the discovery of different stem cells and the potential therapies based on these cells. The genesis of stem cells is followed by laboratory steps of controlled stem cell culturing and derivation. Quality control and teratoma formation assays are important procedures in assessing the properties of the stem cells tested. Derivation methods and the utilization of culturing media are crucial to set proper environmental conditions for controlled differentiation. Among many types of stem tissue applications, the use of graphene scaffolds and the potential of extracellular vesicle-based therapies require attention due to their versatility. The review is summarized by challenges that stem cell therapy must overcome to be accepted worldwide. A wide variety of possibilities makes this cutting edge therapy a turning point in modern medicine, providing hope for untreatable diseases.
Stem cell classification
Stem cells are unspecialized cells of the human body. They are able to differentiate into any cell of an organism and have the ability of self-renewal. Stem cells exist both in embryos and adult cells. There are several steps of specialization. Developmental potency is reduced with each step, which means that a unipotent stem cell is not able to differentiate into as many types of cells as a pluripotent one. This chapter will focus on stem cell classification to make it easier for the reader to comprehend the following chapters.
Totipotent stem cells are able to divide and differentiate into cells of the whole organism. Totipotency has the highest differentiation potential and allows cells to form both embryo and extra-embryonic structures. One example of a totipotent cell is a zygote, which is formed after a sperm fertilizes an egg. These cells can later develop either into any of the three germ layers or form a placenta. After approximately 4 days, the blastocyst’s inner cell mass becomes pluripotent. This structure is the source of pluripotent cells.
Pluripotent stem cells (PSCs) form cells of all germ layers but not extraembryonic structures, such as the placenta. Embryonic stem cells (ESCs) are an example. ESCs are derived from the inner cell mass of preimplantation embryos. Another example is induced pluripotent stem cells (iPSCs) derived from the epiblast layer of implanted embryos. Their pluripotency is a continuum, starting from completely pluripotent cells such as ESCs and iPSCs and ending on representatives with less potency—multi-, oligo- or unipotent cells. One of the methods to assess their activity and spectrum is the teratoma formation assay. iPSCs are artificially generated from somatic cells, and they function similarly to PSCs. Their culturing and utilization are very promising for present and future regenerative medicine.
Multipotent stem cells have a narrower spectrum of differentiation than PSCs, but they can specialize in discrete cells of specific cell lineages. One example is a haematopoietic stem cell, which can develop into several types of blood cells. After differentiation, a haematopoietic stem cell becomes an oligopotent cell. Its differentiation abilities are then restricted to cells of its lineage. However, some multipotent cells are capable of conversion into unrelated cell types, which suggests naming them pluripotent cells.
Oligopotent stem cells can differentiate into several cell types. A myeloid stem cell is an example that can divide into white blood cells but not red blood cells.
Unipotent stem cells are characterized by the narrowest differentiation capabilities and a special property of dividing repeatedly. Their latter feature makes them a promising candidate for therapeutic use in regenerative medicine. These cells are only able to form one cell type, e.g. dermatocytes.
Stem cell biology
A blastocyst is formed after the fusion of sperm and ovum fertilization. Its inner wall is lined with short-lived stem cells, namely, embryonic stem cells. Blastocysts are composed of two distinct cell types: the inner cell mass (ICM), which develops into epiblasts and induces the development of a foetus, and the trophectoderm (TE). Blastocysts are responsible for the regulation of the ICM microenvironment. The TE continues to develop and forms the extraembryonic support structures needed for the successful origin of the embryo, such as the placenta. As the TE begins to form a specialized support structure, the ICM cells remain undifferentiated, fully pluripotent and proliferative [ 1 ]. The pluripotency of stem cells allows them to form any cell of the organism. Human embryonic stem cells (hESCs) are derived from the ICM. During the process of embryogenesis, cells form aggregations called germ layers: endoderm, mesoderm and ectoderm (Fig. 1 ), each eventually giving rise to differentiated cells and tissues of the foetus and, later on, the adult organism [ 2 ]. After hESCs differentiate into one of the germ layers, they become multipotent stem cells, whose potency is limited to only the cells of the germ layer. This process is short in human development. After that, pluripotent stem cells occur all over the organism as undifferentiated cells, and their key abilities are proliferation by the formation of the next generation of stem cells and differentiation into specialized cells under certain physiological conditions.
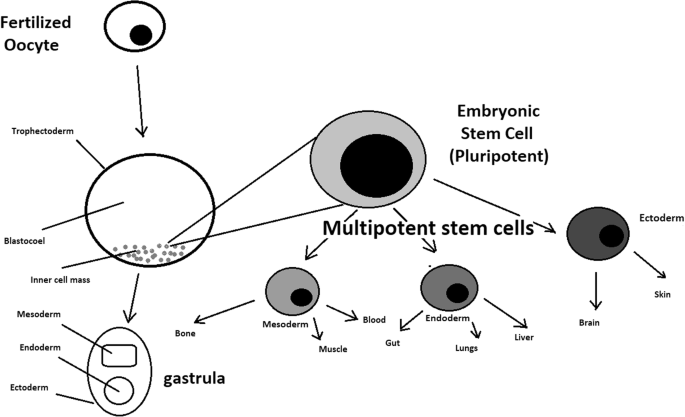
Oocyte development and formation of stem cells: the blastocoel, which is formed from oocytes, consists of embryonic stem cells that later differentiate into mesodermal, ectodermal, or endodermal cells. Blastocoel develops into the gastrula
Signals that influence the stem cell specialization process can be divided into external, such as physical contact between cells or chemical secretion by surrounding tissue, and internal, which are signals controlled by genes in DNA.
Stem cells also act as internal repair systems of the body. The replenishment and formation of new cells are unlimited as long as an organism is alive. Stem cell activity depends on the organ in which they are in; for example, in bone marrow, their division is constant, although in organs such as the pancreas, division only occurs under special physiological conditions.
Stem cell functional division
Whole-body development.
During division, the presence of different stem cells depends on organism development. Somatic stem cell ESCs can be distinguished. Although the derivation of ESCs without separation from the TE is possible, such a combination has growth limits. Because proliferating actions are limited, co-culture of these is usually avoided.
ESCs are derived from the inner cell mass of the blastocyst, which is a stage of pre-implantation embryo ca. 4 days after fertilization. After that, these cells are placed in a culture dish filled with culture medium. Passage is an inefficient but popular process of sub-culturing cells to other dishes. These cells can be described as pluripotent because they are able to eventually differentiate into every cell type in the organism. Since the beginning of their studies, there have been ethical restrictions connected to the medical use of ESCs in therapies. Most embryonic stem cells are developed from eggs that have been fertilized in an in vitro clinic, not from eggs fertilized in vivo.
Somatic or adult stem cells are undifferentiated and found among differentiated cells in the whole body after development. The function of these cells is to enable the healing, growth, and replacement of cells that are lost each day. These cells have a restricted range of differentiation options. Among many types, there are the following:
Mesenchymal stem cells are present in many tissues. In bone marrow, these cells differentiate mainly into the bone, cartilage, and fat cells. As stem cells, they are an exception because they act pluripotently and can specialize in the cells of any germ layer.
Neural cells give rise to nerve cells and their supporting cells—oligodendrocytes and astrocytes.
Haematopoietic stem cells form all kinds of blood cells: red, white, and platelets.
Skin stem cells form, for example, keratinocytes, which form a protective layer of skin.
The proliferation time of somatic stem cells is longer than that of ESCs. It is possible to reprogram adult stem cells back to their pluripotent state. This can be performed by transferring the adult nucleus into the cytoplasm of an oocyte or by fusion with the pluripotent cell. The same technique was used during cloning of the famous Dolly sheep.
hESCs are involved in whole-body development. They can differentiate into pluripotent, totipotent, multipotent, and unipotent cells (Fig. 2 ) [ 2 ].
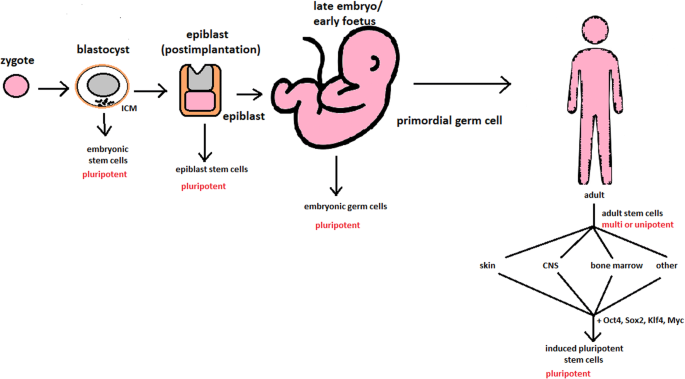
Changes in the potency of stem cells in human body development. Potency ranges from pluripotent cells of the blastocyst to unipotent cells of a specific tissue in a human body such as the skin, CNS, or bone marrow. Reversed pluripotency can be achieved by the formation of induced pluripotent stem cells using either octamer-binding transcription factor (Oct4), sex-determining region Y (Sox2), Kruppel-like factor 4 (Klf4), or the Myc gene
Pluripotent cells can be named totipotent if they can additionally form extraembryonic tissues of the embryo. Multipotent cells are restricted in differentiating to each cell type of given tissue. When tissue contains only one lineage of cells, stem cells that form them are called either called oligo- or unipotent.
iPSC quality control and recognition by morphological differences
The comparability of stem cell lines from different individuals is needed for iPSC lines to be used in therapeutics [ 3 ]. Among critical quality procedures, the following can be distinguished:
Short tandem repeat analysis—This is the comparison of specific loci on the DNA of the samples. It is used in measuring an exact number of repeating units. One unit consists of 2 to 13 nucleotides repeating many times on the DNA strand. A polymerase chain reaction is used to check the lengths of short tandem repeats. The genotyping procedure of source tissue, cells, and iPSC seed and master cell banks is recommended.
Identity analysis—The unintentional switching of lines, resulting in other stem cell line contamination, requires rigorous assay for cell line identification.
Residual vector testing—An appearance of reprogramming vectors integrated into the host genome is hazardous, and testing their presence is a mandatory procedure. It is a commonly used procedure for generating high-quality iPSC lines. An acceptable threshold in high-quality research-grade iPSC line collections is ≤ 1 plasmid copies per 100 cells. During the procedure, 2 different regions, common to all plasmids, should be used as specific targets, such as EBNA and CAG sequences [ 3 ]. To accurately represent the test reactions, a standard curve needs to be prepared in a carrier of gDNA from a well-characterized hPSC line. For calculations of plasmid copies per cell, it is crucial to incorporate internal reference gDNA sequences to allow the quantification of, for example, ribonuclease P (RNaseP) or human telomerase reverse transcriptase (hTERT).
Karyotype—A long-term culture of hESCs can accumulate culture-driven mutations [ 4 ]. Because of that, it is crucial to pay additional attention to genomic integrity. Karyotype tests can be performed by resuscitating representative aliquots and culturing them for 48–72 h before harvesting cells for karyotypic analysis. If abnormalities are found within the first 20 karyotypes, the analysis must be repeated on a fresh sample. When this situation is repeated, the line is evaluated as abnormal. Repeated abnormalities must be recorded. Although karyology is a crucial procedure in stem cell quality control, the single nucleotide polymorphism (SNP) array, discussed later, has approximately 50 times higher resolution.
Viral testing—When assessing the quality of stem cells, all tests for harmful human adventitious agents must be performed (e.g. hepatitis C or human immunodeficiency virus). This procedure must be performed in the case of non-xeno-free culture agents.
Bacteriology—Bacterial or fungal sterility tests can be divided into culture- or broth-based tests. All the procedures must be recommended by pharmacopoeia for the jurisdiction in which the work is performed.
Single nucleotide polymorphism arrays—This procedure is a type of DNA microarray that detects population polymorphisms by enabling the detection of subchromosomal changes and the copy-neutral loss of heterozygosity, as well as an indication of cellular transformation. The SNP assay consists of three components. The first is labelling fragmented nucleic acid sequences with fluorescent dyes. The second is an array that contains immobilized allele-specific oligonucleotide (ASO) probes. The last component detects, records, and eventually interprets the signal.
Flow cytometry—This is a technique that utilizes light to count and profile cells in a heterogeneous fluid mixture. It allows researchers to accurately and rapidly collect data from heterogeneous fluid mixtures with live cells. Cells are passed through a narrow channel one by one. During light illumination, sensors detect light emitted or refracted from the cells. The last step is data analysis, compilation and integration into a comprehensive picture of the sample.
Phenotypic pluripotency assays—Recognizing undifferentiated cells is crucial in successful stem cell therapy. Among other characteristics, stem cells appear to have a distinct morphology with a high nucleus to cytoplasm ratio and a prominent nucleolus. Cells appear to be flat with defined borders, in contrast to differentiating colonies, which appear as loosely located cells with rough borders [ 5 ]. It is important that images of ideal and poor quality colonies for each cell line are kept in laboratories, so whenever there is doubt about the quality of culture, it can always be checked according to the representative image. Embryoid body formation or directed differentiation of monolayer cultures to produce cell types representative of all three embryonic germ layers must be performed. It is important to note that colonies cultured under different conditions may have different morphologies [ 6 ].
Histone modification and DNA methylation—Quality control can be achieved by using epigenetic analysis tools such as histone modification or DNA methylation. When stem cells differentiate, the methylation process silences pluripotency genes, which reduces differentiation potential, although other genes may undergo demethylation to become expressed [ 7 ]. It is important to emphasize that stem cell identity, together with its morphological characteristics, is also related to its epigenetic profile [ 8 , 9 ]. According to Brindley [ 10 ], there is a relationship between epigenetic changes, pluripotency, and cell expansion conditions, which emphasizes that unmethylated regions appear to be serum-dependent.
hESC derivation and media
hESCs can be derived using a variety of methods, from classic culturing to laser-assisted methodologies or microsurgery [ 11 ]. hESC differentiation must be specified to avoid teratoma formation (see Fig. 3 ).
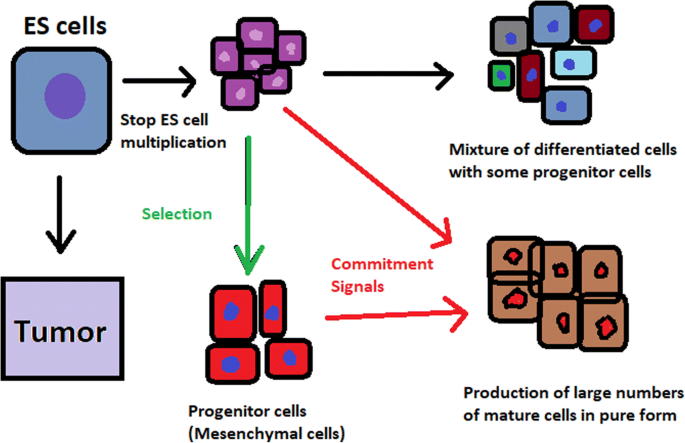
Spontaneous differentiation of hESCs causes the formation of a heterogeneous cell population. There is a different result, however, when commitment signals (in forms of soluble factors and culture conditions) are applied and enable the selection of progenitor cells
hESCs spontaneously differentiate into embryonic bodies (EBs) [ 12 ]. EBs can be studied instead of embryos or animals to predict their effects on early human development. There are many different methods for acquiring EBs, such as bioreactor culture [ 13 ], hanging drop culture [ 12 ], or microwell technology [ 14 , 15 ]. These methods allow specific precursors to form in vitro [ 16 ].
The essential part of these culturing procedures is a separation of inner cell mass to culture future hESCs (Fig. 4 ) [ 17 ]. Rosowski et al. [ 18 ] emphasizes that particular attention must be taken in controlling spontaneous differentiation. When the colony reaches the appropriate size, cells must be separated. The occurrence of pluripotent cells lasts for 1–2 days. Because the classical utilization of hESCs caused ethical concerns about gastrulas used during procedures, Chung et al. [ 19 ] found out that it is also possible to obtain hESCs from four cell embryos, leaving a higher probability of embryo survival. Additionally, Zhang et al. [ 20 ] used only in vitro fertilization growth-arrested cells.
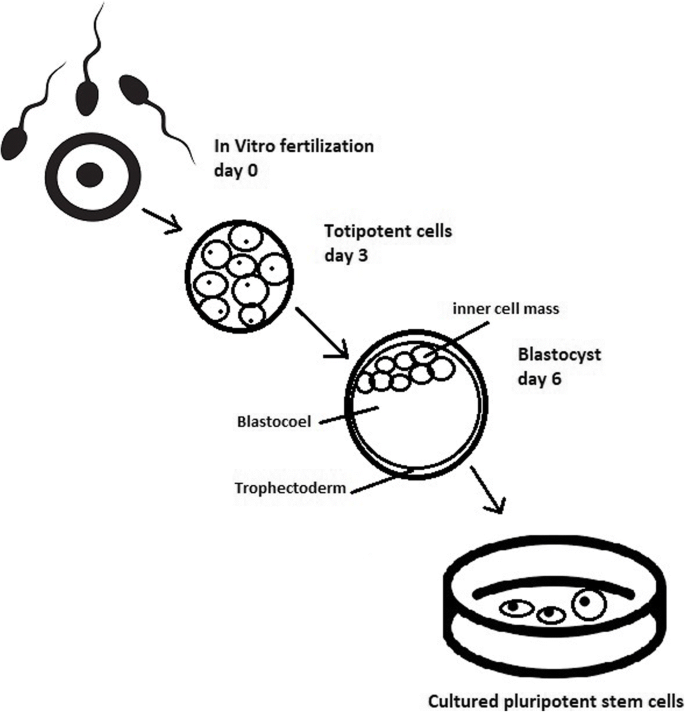
Culturing of pluripotent stem cells in vitro. Three days after fertilization, totipotent cells are formed. Blastocysts with ICM are formed on the sixth day after fertilization. Pluripotent stem cells from ICM can then be successfully transmitted on a dish
Cell passaging is used to form smaller clusters of cells on a new culture surface [ 21 ]. There are four important passaging procedures.
Enzymatic dissociation is a cutting action of enzymes on proteins and adhesion domains that bind the colony. It is a gentler method than the manual passage. It is crucial to not leave hESCs alone after passaging. Solitary cells are more sensitive and can easily undergo cell death; collagenase type IV is an example [ 22 , 23 ].
Manual passage , on the other hand, focuses on using cell scratchers. The selection of certain cells is not necessary. This should be done in the early stages of cell line derivation [ 24 ].
Trypsin utilization allows a healthy, automated hESC passage. Good Manufacturing Practice (GMP)-grade recombinant trypsin is widely available in this procedure [ 24 ]. However, there is a risk of decreasing the pluripotency and viability of stem cells [ 25 ]. Trypsin utilization can be halted with an inhibitor of the protein rho-associated protein kinase (ROCK) [ 26 ].
Ethylenediaminetetraacetic acid ( EDTA ) indirectly suppresses cell-to-cell connections by chelating divalent cations. Their suppression promotes cell dissociation [ 27 ].
Stem cells require a mixture of growth factors and nutrients to differentiate and develop. The medium should be changed each day.
Traditional culture methods used for hESCs are mouse embryonic fibroblasts (MEFs) as a feeder layer and bovine serum [ 28 ] as a medium. Martin et al. [ 29 ] demonstrated that hESCs cultured in the presence of animal products express the non-human sialic acid, N -glycolylneuraminic acid (NeuGc). Feeder layers prevent uncontrolled proliferation with factors such as leukaemia inhibitory factor (LIF) [ 30 ].
First feeder layer-free culture can be supplemented with serum replacement, combined with laminin [ 31 ]. This causes stable karyotypes of stem cells and pluripotency lasting for over a year.
Initial culturing media can be serum (e.g. foetal calf serum FCS), artificial replacement such as synthetic serum substitute (SSS), knockout serum replacement (KOSR), or StemPro [ 32 ]. The simplest culture medium contains only eight essential elements: DMEM/F12 medium, selenium, NaHCO 3, l -ascorbic acid, transferrin, insulin, TGFβ1, and FGF2 [ 33 ]. It is not yet fully known whether culture systems developed for hESCs can be allowed without adaptation in iPSC cultures.
Turning point in stem cell therapy
The turning point in stem cell therapy appeared in 2006, when scientists Shinya Yamanaka, together with Kazutoshi Takahashi, discovered that it is possible to reprogram multipotent adult stem cells to the pluripotent state. This process avoided endangering the foetus’ life in the process. Retrovirus-mediated transduction of mouse fibroblasts with four transcription factors (Oct-3/4, Sox2, KLF4, and c-Myc) [ 34 ] that are mainly expressed in embryonic stem cells could induce the fibroblasts to become pluripotent (Fig. 5 ) [ 35 ]. This new form of stem cells was named iPSCs. One year later, the experiment also succeeded with human cells [ 36 ]. After this success, the method opened a new field in stem cell research with a generation of iPSC lines that can be customized and biocompatible with the patient. Recently, studies have focused on reducing carcinogenesis and improving the conduction system.
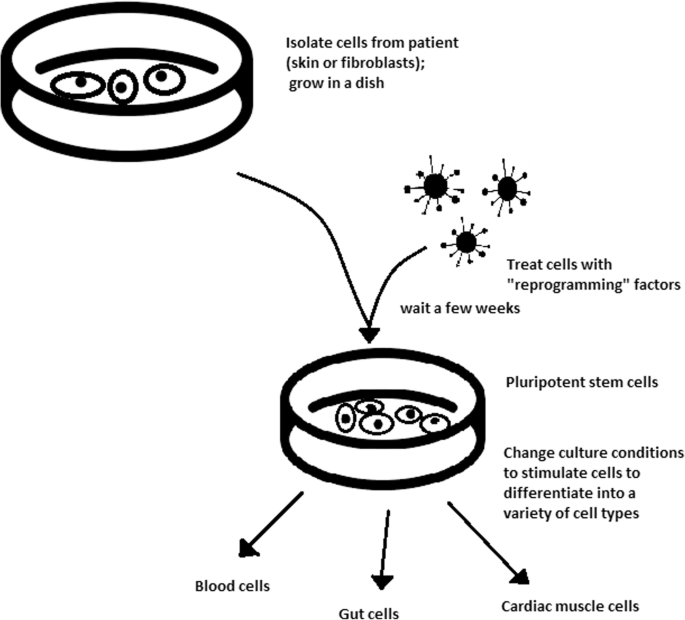
Retroviral-mediated transduction induces pluripotency in isolated patient somatic cells. Target cells lose their role as somatic cells and, once again, become pluripotent and can differentiate into any cell type of human body
The turning point was influenced by former discoveries that happened in 1962 and 1987.
The former discovery was about scientist John Gurdon successfully cloning frogs by transferring a nucleus from a frog’s somatic cells into an oocyte. This caused a complete reversion of somatic cell development [ 37 ]. The results of his experiment became an immense discovery since it was previously believed that cell differentiation is a one-way street only, but his experiment suggested the opposite and demonstrated that it is even possible for a somatic cell to again acquire pluripotency [ 38 ].
The latter was a discovery made by Davis R.L. that focused on fibroblast DNA subtraction. Three genes were found that originally appeared in myoblasts. The enforced expression of only one of the genes, named myogenic differentiation 1 (Myod1), caused the conversion of fibroblasts into myoblasts, showing that reprogramming cells is possible, and it can even be used to transform cells from one lineage to another [ 39 ].
Although pluripotency can occur naturally only in embryonic stem cells, it is possible to induce terminally differentiated cells to become pluripotent again. The process of direct reprogramming converts differentiated somatic cells into iPSC lines that can form all cell types of an organism. Reprogramming focuses on the expression of oncogenes such as Myc and Klf4 (Kruppel-like factor 4). This process is enhanced by a downregulation of genes promoting genome stability, such as p53. Additionally, cell reprogramming involves histone alteration. All these processes can cause potential mutagenic risk and later lead to an increased number of mutations. Quinlan et al. [ 40 ] checked fully pluripotent mouse iPSCs using whole genome DNA sequencing and structural variation (SV) detection algorithms. Based on those studies, it was confirmed that although there were single mutations in the non-genetic region, there were non-retrotransposon insertions. This led to the conclusion that current reprogramming methods can produce fully pluripotent iPSCs without severe genomic alterations.
During the course of development from pluripotent hESCs to differentiated somatic cells, crucial changes appear in the epigenetic structure of these cells. There is a restriction or permission of the transcription of genes relevant to each cell type. When somatic cells are being reprogrammed using transcription factors, all the epigenetic architecture has to be reconditioned to achieve iPSCs with pluripotency [ 41 ]. However, cells of each tissue undergo specific somatic genomic methylation. This influences transcription, which can further cause alterations in induced pluripotency [ 42 ].
Source of iPSCs
Because pluripotent cells can propagate indefinitely and differentiate into any kind of cell, they can be an unlimited source, either for replacing lost or diseased tissues. iPSCs bypass the need for embryos in stem cell therapy. Because they are made from the patient’s own cells, they are autologous and no longer generate any risk of immune rejection.
At first, fibroblasts were used as a source of iPSCs. Because a biopsy was needed to achieve these types of cells, the technique underwent further research. Researchers investigated whether more accessible cells could be used in the method. Further, other cells were used in the process: peripheral blood cells, keratinocytes, and renal epithelial cells found in urine. An alternative strategy to stem cell transplantation can be stimulating a patient’s endogenous stem cells to divide or differentiate, occurring naturally when skin wounds are healing. In 2008, pancreatic exocrine cells were shown to be reprogrammed to functional, insulin-producing beta cells [ 43 ].
The best stem cell source appears to be the fibroblasts, which is more tempting in the case of logistics since its stimulation can be fast and better controlled [ 44 ].
- Teratoma formation assay
The self-renewal and differentiation capabilities of iPSCs have gained significant interest and attention in regenerative medicine sciences. To study their abilities, a quality-control assay is needed, of which one of the most important is the teratoma formation assay. Teratomas are benign tumours. Teratomas are capable of rapid growth in vivo and are characteristic because of their ability to develop into tissues of all three germ layers simultaneously. Because of the high pluripotency of teratomas, this formation assay is considered an assessment of iPSC’s abilities [ 45 ].
Teratoma formation rate, for instance, was observed to be elevated in human iPSCs compared to that in hESCs [ 46 ]. This difference may be connected to different differentiation methods and cell origins. Most commonly, the teratoma assay involves an injection of examined iPSCs subcutaneously or under the testis or kidney capsule in mice, which are immune-deficient [ 47 ]. After injection, an immature but recognizable tissue can be observed, such as the kidney tubules, bone, cartilage, or neuroepithelium [ 30 ]. The injection site may have an impact on the efficiency of teratoma formation [ 48 ].
There are three groups of markers used in this assay to differentiate the cells of germ layers. For endodermal tissue, there is insulin/C-peptide and alpha-1 antitrypsin [ 49 ]. For the mesoderm, derivatives can be used, e.g. cartilage matrix protein for the bone and alcian blue for the cartilage. As ectodermal markers, class III B botulin or keratin can be used for keratinocytes.
Teratoma formation assays are considered the gold standard for demonstrating the pluripotency of human iPSCs, demonstrating their possibilities under physiological conditions. Due to their actual tissue formation, they could be used for the characterization of many cell lineages [ 50 ].
Directed differentiation
To be useful in therapy, stem cells must be converted into desired cell types as necessary or else the whole regenerative medicine process will be pointless. Differentiation of ESCs is crucial because undifferentiated ESCs can cause teratoma formation in vivo. Understanding and using signalling pathways for differentiation is an important method in successful regenerative medicine. In directed differentiation, it is likely to mimic signals that are received by cells when they undergo successive stages of development [ 51 ]. The extracellular microenvironment plays a significant role in controlling cell behaviour. By manipulating the culture conditions, it is possible to restrict specific differentiation pathways and generate cultures that are enriched in certain precursors in vitro. However, achieving a similar effect in vivo is challenging. It is crucial to develop culture conditions that will allow the promotion of homogenous and enhanced differentiation of ESCs into functional and desired tissues.
Regarding the self-renewal of embryonic stem cells, Hwang et al. [ 52 ] noted that the ideal culture method for hESC-based cell and tissue therapy would be a defined culture free of either the feeder layer or animal components. This is because cell and tissue therapy requires the maintenance of large quantities of undifferentiated hESCs, which does not make feeder cells suitable for such tasks.
Most directed differentiation protocols are formed to mimic the development of an inner cell mass during gastrulation. During this process, pluripotent stem cells differentiate into ectodermal, mesodermal, or endodermal progenitors. Mall molecules or growth factors induce the conversion of stem cells into appropriate progenitor cells, which will later give rise to the desired cell type. There is a variety of signal intensities and molecular families that may affect the establishment of germ layers in vivo, such as fibroblast growth factors (FGFs) [ 53 ]; the Wnt family [ 54 ] or superfamily of transforming growth factors—β(TGFβ); and bone morphogenic proteins (BMP) [ 55 ]. Each candidate factor must be tested on various concentrations and additionally applied to various durations because the precise concentrations and times during which developing cells in embryos are influenced during differentiation are unknown. For instance, molecular antagonists of endogenous BMP and Wnt signalling can be used for ESC formation of ectoderm [ 56 ]. However, transient Wnt and lower concentrations of the TGFβ family trigger mesodermal differentiation [ 57 ]. Regarding endoderm formation, a higher activin A concentration may be required [ 58 , 59 ].
There are numerous protocols about the methods of forming progenitors of cells of each of germ layers, such as cardiomyocytes [ 60 ], hepatocytes [ 61 ], renal cells [ 62 ], lung cells [ 63 , 64 ], motor neurons [ 65 ], intestinal cells [ 66 ], or chondrocytes [ 67 ].
Directed differentiation of either iPSCs or ESCs into, e.g. hepatocytes, could influence and develop the study of the molecular mechanisms in human liver development. In addition, it could also provide the possibility to form exogenous hepatocytes for drug toxicity testing [ 68 ].
Levels of concentration and duration of action with a specific signalling molecule can cause a variety of factors. Unfortunately, for now, a high cost of recombinant factors is likely to limit their use on a larger scale in medicine. The more promising technique focuses on the use of small molecules. These can be used for either activating or deactivating specific signalling pathways. They enhance reprogramming efficiency by creating cells that are compatible with the desired type of tissue. It is a cheaper and non-immunogenic method.
One of the successful examples of small-molecule cell therapies is antagonists and agonists of the Hedgehog pathway. They show to be very useful in motor neuron regeneration [ 69 ]. Endogenous small molecules with their function in embryonic development can also be used in in vitro methods to induce the differentiation of cells; for example, retinoic acid, which is responsible for patterning the nervous system in vivo [ 70 ], surprisingly induced retinal cell formation when the laboratory procedure involved hESCs [ 71 ].
The efficacy of differentiation factors depends on functional maturity, efficiency, and, finally, introducing produced cells to their in vivo equivalent. Topography, shear stress, and substrate rigidity are factors influencing the phenotype of future cells [ 72 ].
The control of biophysical and biochemical signals, the biophysical environment, and a proper guide of hESC differentiation are important factors in appropriately cultured stem cells.
Stem cell utilization and their manufacturing standards and culture systems
The European Medicines Agency and the Food and Drug Administration have set Good Manufacturing Practice (GMP) guidelines for safe and appropriate stem cell transplantation. In the past, protocols used for stem cell transplantation required animal-derived products [ 73 ].
The risk of introducing animal antigens or pathogens caused a restriction in their use. Due to such limitations, the technique required an obvious update [ 74 ]. Now, it is essential to use xeno-free equivalents when establishing cell lines that are derived from fresh embryos and cultured from human feeder cell lines [ 75 ]. In this method, it is crucial to replace any non-human materials with xeno-free equivalents [ 76 ].
NutriStem with LN-511, TeSR2 with human recombinant laminin (LN-511), and RegES with human foreskin fibroblasts (HFFs) are commonly used xeno-free culture systems [ 33 ]. There are many organizations and international initiatives, such as the National Stem Cell Bank, that provide stem cell lines for treatment or medical research [ 77 ].

Stem cell use in medicine
Stem cells have great potential to become one of the most important aspects of medicine. In addition to the fact that they play a large role in developing restorative medicine, their study reveals much information about the complex events that happen during human development.
The difference between a stem cell and a differentiated cell is reflected in the cells’ DNA. In the former cell, DNA is arranged loosely with working genes. When signals enter the cell and the differentiation process begins, genes that are no longer needed are shut down, but genes required for the specialized function will remain active. This process can be reversed, and it is known that such pluripotency can be achieved by interaction in gene sequences. Takahashi and Yamanaka [ 78 ] and Loh et al. [ 79 ] discovered that octamer-binding transcription factor 3 and 4 (Oct3/4), sex determining region Y (SRY)-box 2 and Nanog genes function as core transcription factors in maintaining pluripotency. Among them, Oct3/4 and Sox2 are essential for the generation of iPSCs.
Many serious medical conditions, such as birth defects or cancer, are caused by improper differentiation or cell division. Currently, several stem cell therapies are possible, among which are treatments for spinal cord injury, heart failure [ 80 ], retinal and macular degeneration [ 81 ], tendon ruptures, and diabetes type 1 [ 82 ]. Stem cell research can further help in better understanding stem cell physiology. This may result in finding new ways of treating currently incurable diseases.
Haematopoietic stem cell transplantation
Haematopoietic stem cells are important because they are by far the most thoroughly characterized tissue-specific stem cell; after all, they have been experimentally studied for more than 50 years. These stem cells appear to provide an accurate paradigm model system to study tissue-specific stem cells, and they have potential in regenerative medicine.
Multipotent haematopoietic stem cell (HSC) transplantation is currently the most popular stem cell therapy. Target cells are usually derived from the bone marrow, peripheral blood, or umbilical cord blood [ 83 ]. The procedure can be autologous (when the patient’s own cells are used), allogenic (when the stem cell comes from a donor), or syngeneic (from an identical twin). HSCs are responsible for the generation of all functional haematopoietic lineages in blood, including erythrocytes, leukocytes, and platelets. HSC transplantation solves problems that are caused by inappropriate functioning of the haematopoietic system, which includes diseases such as leukaemia and anaemia. However, when conventional sources of HSC are taken into consideration, there are some important limitations. First, there is a limited number of transplantable cells, and an efficient way of gathering them has not yet been found. There is also a problem with finding a fitting antigen-matched donor for transplantation, and viral contamination or any immunoreactions also cause a reduction in efficiency in conventional HSC transplantations. Haematopoietic transplantation should be reserved for patients with life-threatening diseases because it has a multifactorial character and can be a dangerous procedure. iPSC use is crucial in this procedure. The use of a patient’s own unspecialized somatic cells as stem cells provides the greatest immunological compatibility and significantly increases the success of the procedure.
Stem cells as a target for pharmacological testing
Stem cells can be used in new drug tests. Each experiment on living tissue can be performed safely on specific differentiated cells from pluripotent cells. If any undesirable effect appears, drug formulas can be changed until they reach a sufficient level of effectiveness. The drug can enter the pharmacological market without harming any live testers. However, to test the drugs properly, the conditions must be equal when comparing the effects of two drugs. To achieve this goal, researchers need to gain full control of the differentiation process to generate pure populations of differentiated cells.
Stem cells as an alternative for arthroplasty
One of the biggest fears of professional sportsmen is getting an injury, which most often signifies the end of their professional career. This applies especially to tendon injuries, which, due to current treatment options focusing either on conservative or surgical treatment, often do not provide acceptable outcomes. Problems with the tendons start with their regeneration capabilities. Instead of functionally regenerating after an injury, tendons merely heal by forming scar tissues that lack the functionality of healthy tissues. Factors that may cause this failed healing response include hypervascularization, deposition of calcific materials, pain, or swelling [ 84 ].
Additionally, in addition to problems with tendons, there is a high probability of acquiring a pathological condition of joints called osteoarthritis (OA) [ 85 ]. OA is common due to the avascular nature of articular cartilage and its low regenerative capabilities [ 86 ]. Although arthroplasty is currently a common procedure in treating OA, it is not ideal for younger patients because they can outlive the implant and will require several surgical procedures in the future. These are situations where stem cell therapy can help by stopping the onset of OA [ 87 ]. However, these procedures are not well developed, and the long-term maintenance of hyaline cartilage requires further research.
Osteonecrosis of the femoral hip (ONFH) is a refractory disease associated with the collapse of the femoral head and risk of hip arthroplasty in younger populations [ 88 ]. Although total hip arthroplasty (THA) is clinically successful, it is not ideal for young patients, mostly due to the limited lifetime of the prosthesis. An increasing number of clinical studies have evaluated the therapeutic effect of stem cells on ONFH. Most of the authors demonstrated positive outcomes, with reduced pain, improved function, or avoidance of THA [ 89 , 90 , 91 ].
Rejuvenation by cell programming
Ageing is a reversible epigenetic process. The first cell rejuvenation study was published in 2011 [ 92 ]. Cells from aged individuals have different transcriptional signatures, high levels of oxidative stress, dysfunctional mitochondria, and shorter telomeres than in young cells [ 93 ]. There is a hypothesis that when human or mouse adult somatic cells are reprogrammed to iPSCs, their epigenetic age is virtually reset to zero [ 94 ]. This was based on an epigenetic model, which explains that at the time of fertilization, all marks of parenteral ageing are erased from the zygote’s genome and its ageing clock is reset to zero [ 95 ].
In their study, Ocampo et al. [ 96 ] used Oct4, Sox2, Klf4, and C-myc genes (OSKM genes) and affected pancreas and skeletal muscle cells, which have poor regenerative capacity. Their procedure revealed that these genes can also be used for effective regenerative treatment [ 97 ]. The main challenge of their method was the need to employ an approach that does not use transgenic animals and does not require an indefinitely long application. The first clinical approach would be preventive, focused on stopping or slowing the ageing rate. Later, progressive rejuvenation of old individuals can be attempted. In the future, this method may raise some ethical issues, such as overpopulation, leading to lower availability of food and energy.
For now, it is important to learn how to implement cell reprogramming technology in non-transgenic elder animals and humans to erase marks of ageing without removing the epigenetic marks of cell identity.
Cell-based therapies
Stem cells can be induced to become a specific cell type that is required to repair damaged or destroyed tissues (Fig. 6 ). Currently, when the need for transplantable tissues and organs outweighs the possible supply, stem cells appear to be a perfect solution for the problem. The most common conditions that benefit from such therapy are macular degenerations [ 98 ], strokes [ 99 ], osteoarthritis [ 89 , 90 ], neurodegenerative diseases, and diabetes [ 100 ]. Due to this technique, it can become possible to generate healthy heart muscle cells and later transplant them to patients with heart disease.
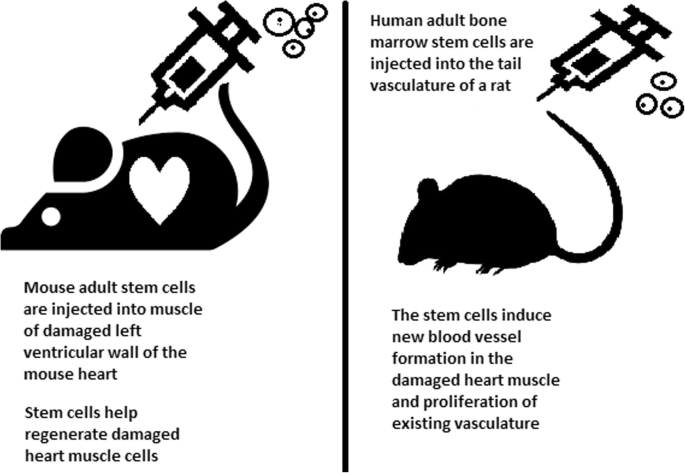
Stem cell experiments on animals. These experiments are one of the many procedures that proved stem cells to be a crucial factor in future regenerative medicine
In the case of type 1 diabetes, insulin-producing cells in the pancreas are destroyed due to an autoimmunological reaction. As an alternative to transplantation therapy, it can be possible to induce stem cells to differentiate into insulin-producing cells [ 101 ].
Stem cells and tissue banks
iPS cells with their theoretically unlimited propagation and differentiation abilities are attractive for the present and future sciences. They can be stored in a tissue bank to be an essential source of human tissue used for medical examination. The problem with conventional differentiated tissue cells held in the laboratory is that their propagation features diminish after time. This does not occur in iPSCs.
The umbilical cord is known to be rich in mesenchymal stem cells. Due to its cryopreservation immediately after birth, its stem cells can be successfully stored and used in therapies to prevent the future life-threatening diseases of a given patient.
Stem cells of human exfoliated deciduous teeth (SHED) found in exfoliated deciduous teeth has the ability to develop into more types of body tissues than other stem cells [ 102 ] (Table 1 ). Techniques of their collection, isolation, and storage are simple and non-invasive. Among the advantages of banking, SHED cells are:
Guaranteed donor-match autologous transplant that causes no immune reaction and rejection of cells [ 103 ]
Simple and painless for both child and parent
Less than one third of the cost of cord blood storage
Not subject to the same ethical concerns as embryonic stem cells [ 104 ]
In contrast to cord blood stem cells, SHED cells are able to regenerate into solid tissues such as connective, neural, dental, or bone tissue [ 105 , 106 ]
SHED can be useful for close relatives of the donor
Fertility diseases
In 2011, two researchers, Katsuhiko Hayashi et al. [ 107 ], showed in an experiment on mice that it is possible to form sperm from iPSCs. They succeeded in delivering healthy and fertile pups in infertile mice. The experiment was also successful for female mice, where iPSCs formed fully functional eggs .
Young adults at risk of losing their spermatogonial stem cells (SSC), mostly cancer patients, are the main target group that can benefit from testicular tissue cryopreservation and autotransplantation. Effective freezing methods for adult and pre-pubertal testicular tissue are available [ 108 ].
Qiuwan et al. [ 109 ] provided important evidence that human amniotic epithelial cell (hAEC) transplantation could effectively improve ovarian function by inhibiting cell apoptosis and reducing inflammation in injured ovarian tissue of mice, and it could be a promising strategy for the management of premature ovarian failure or insufficiency in female cancer survivors.
For now, reaching successful infertility treatments in humans appears to be only a matter of time, but there are several challenges to overcome. First, the process needs to have high efficiency; second, the chances of forming tumours instead of eggs or sperm must be maximally reduced. The last barrier is how to mature human sperm and eggs in the lab without transplanting them to in vivo conditions, which could cause either a tumour risk or an invasive procedure.
Therapy for incurable neurodegenerative diseases
Thanks to stem cell therapy, it is possible not only to delay the progression of incurable neurodegenerative diseases such as Parkinson’s disease, Alzheimer’s disease (AD), and Huntington disease, but also, most importantly, to remove the source of the problem. In neuroscience, the discovery of neural stem cells (NSCs) has nullified the previous idea that adult CNS were not capable of neurogenesis [ 110 , 111 ]. Neural stem cells are capable of improving cognitive function in preclinical rodent models of AD [ 112 , 113 , 114 ]. Awe et al. [ 115 ] clinically derived relevant human iPSCs from skin punch biopsies to develop a neural stem cell-based approach for treating AD. Neuronal degeneration in Parkinson’s disease (PD) is focal, and dopaminergic neurons can be efficiently generated from hESCs. PD is an ideal disease for iPSC-based cell therapy [ 116 ]. However, this therapy is still in an experimental phase ( https://www.ncbi.nlm.nih.gov/pmc/articles/PMC4539501 /). Brain tissue from aborted foetuses was used on patients with Parkinson’s disease [ 117 ]. Although the results were not uniform, they showed that therapies with pure stem cells are an important and achievable therapy.
Stem cell use in dentistry
Teeth represent a very challenging material for regenerative medicine. They are difficult to recreate because of their function in aspects such as articulation, mastication, or aesthetics due to their complicated structure. Currently, there is a chance for stem cells to become more widely used than synthetic materials. Teeth have a large advantage of being the most natural and non-invasive source of stem cells.
For now, without the use of stem cells, the most common periodontological treatments are either growth factors, grafts, or surgery. For example, there are stem cells in periodontal ligament [ 118 , 119 ], which are capable of differentiating into osteoblasts or cementoblasts, and their functions were also assessed in neural cells [ 120 ]. Tissue engineering is a successful method for treating periodontal diseases. Stem cells of the root apical areas are able to recreate periodontal ligament. One of the possible methods of tissue engineering in periodontology is gene therapy performed using adenoviruses-containing growth factors [ 121 ].
As a result of animal studies, dentin regeneration is an effective process that results in the formation of dentin bridges [ 122 ].
Enamel is more difficult to regenerate than dentin. After the differentiation of ameloblastoma cells into the enamel, the former is destroyed, and reparation is impossible. Medical studies have succeeded in differentiating bone marrow stem cells into ameloblastoma [ 123 ].
Healthy dental tissue has a high amount of regular stem cells, although this number is reduced when tissue is either traumatized or inflamed [ 124 ]. There are several dental stem cell groups that can be isolated (Fig. 7 ).
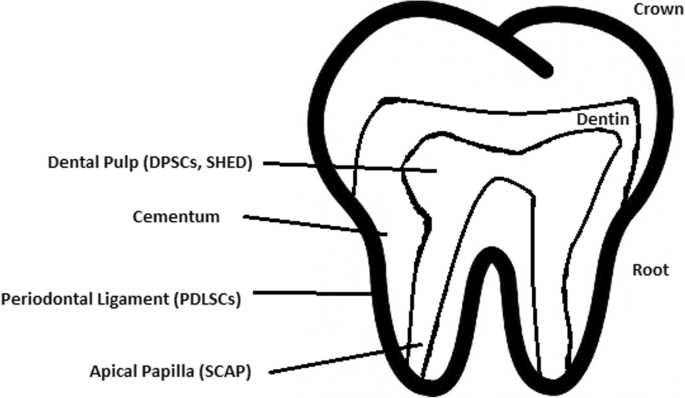
Localization of stem cells in dental tissues. Dental pulp stem cells (DPSCs) and human deciduous teeth stem cells (SHED) are located in the dental pulp. Periodontal ligaments stem cells are located in the periodontal ligament. Apical papilla consists of stem cells from the apical papilla (SCAP)
Dental pulp stem cell (DPSC)
These were the first dental stem cells isolated from the human dental pulp, which were [ 125 ] located inside dental pulp (Table 2 ). They have osteogenic and chondrogenic potential. Mesenchymal stem cells (MSCs) of the dental pulp, when isolated, appear highly clonogenic; they can be isolated from adult tissue (e.g. bone marrow, adipose tissue) and foetal (e.g. umbilical cord) [ 126 ] tissue, and they are able to differentiate densely [ 127 ]. MSCs differentiate into odontoblast-like cells and osteoblasts to form dentin and bone. Their best source locations are the third molars [ 125 ]. DPSCs are the most useful dental source of tissue engineering due to their easy surgical accessibility, cryopreservation possibility, increased production of dentin tissues compared to non-dental stem cells, and their anti-inflammatory abilities. These cells have the potential to be a source for maxillofacial and orthopaedic reconstructions or reconstructions even beyond the oral cavity. DPSCs are able to generate all structures of the developed tooth [ 128 ]. In particular, beneficial results in the use of DPSCs may be achieved when combined with other new therapies, such as periodontal tissue photobiomodulation (laser stimulation), which is an efficient technique in the stimulation of proliferation and differentiation into distinct cell types [ 129 ]. DPSCs can be induced to form neural cells to help treat neurological deficits.
Stem cells of human exfoliated deciduous teeth (SHED) have a faster rate of proliferation than DPSCs and differentiate into an even greater number of cells, e.g. other mesenchymal and non-mesenchymal stem cell derivatives, such as neural cells [ 130 ]. These cells possess one major disadvantage: they form a non-complete dentin/pulp-like complex in vivo. SHED do not undergo the same ethical concerns as embryonic stem cells. Both DPSCs and SHED are able to form bone-like tissues in vivo [ 131 ] and can be used for periodontal, dentin, or pulp regeneration. DPSCs and SHED can be used in treating, for example, neural deficits [ 132 ]. DPSCs alone were tested and successfully applied for alveolar bone and mandible reconstruction [ 133 ].
Periodontal ligament stem cells (PDLSCs)
These cells are used in periodontal ligament or cementum tissue regeneration. They can differentiate into mesenchymal cell lineages to produce collagen-forming cells, adipocytes, cementum tissue, Sharpey’s fibres, and osteoblast-like cells in vitro. PDLSCs exist both on the root and alveolar bone surfaces; however, on the latter, these cells have better differentiation abilities than on the former [ 134 ]. PDLSCs have become the first treatment for periodontal regeneration therapy because of their safety and efficiency [ 135 , 136 ].
Stem cells from apical papilla (SCAP)
These cells are mesenchymal structures located within immature roots. They are isolated from human immature permanent apical papilla. SCAP are the source of odontoblasts and cause apexogenesis. These stem cells can be induced in vitro to form odontoblast-like cells, neuron-like cells, or adipocytes. SCAP have a higher capacity of proliferation than DPSCs, which makes them a better choice for tissue regeneration [ 137 , 138 ].
Dental follicle stem cells (DFCs)
These cells are loose connective tissues surrounding the developing tooth germ. DFCs contain cells that can differentiate into cementoblasts, osteoblasts, and periodontal ligament cells [ 139 , 140 ]. Additionally, these cells proliferate after even more than 30 passages [ 141 ]. DFCs are most commonly extracted from the sac of a third molar. When DFCs are combined with a treated dentin matrix, they can form a root-like tissue with a pulp-dentin complex and eventually form tooth roots [ 141 ]. When DFC sheets are induced by Hertwig’s epithelial root sheath cells, they can produce periodontal tissue; thus, DFCs represent a very promising material for tooth regeneration [ 142 ].
Pulp regeneration in endodontics
Dental pulp stem cells can differentiate into odontoblasts. There are few methods that enable the regeneration of the pulp.
The first is an ex vivo method. Proper stem cells are grown on a scaffold before they are implanted into the root channel [ 143 ].
The second is an in vivo method. This method focuses on injecting stem cells into disinfected root channels after the opening of the in vivo apex. Additionally, the use of a scaffold is necessary to prevent the movement of cells towards other tissues. For now, only pulp-like structures have been created successfully.
Methods of placing stem cells into the root channel constitute are either soft scaffolding [ 144 ] or the application of stem cells in apexogenesis or apexification. Immature teeth are the best source [ 145 ]. Nerve and blood vessel network regeneration are extremely vital to keep pulp tissue healthy.
The potential of dental stem cells is mainly regarding the regeneration of damaged dentin and pulp or the repair of any perforations; in the future, it appears to be even possible to generate the whole tooth. Such an immense success would lead to the gradual replacement of implant treatments. Mandibulary and maxillary defects can be one of the most complicated dental problems for stem cells to address.
Acquiring non-dental tissue cells by dental stem cell differentiation
In 2013, it was reported that it is possible to grow teeth from stem cells obtained extra-orally, e.g. from urine [ 146 ]. Pluripotent stem cells derived from human urine were induced and generated tooth-like structures. The physical properties of the structures were similar to natural ones except for hardness [ 127 ]. Nonetheless, it appears to be a very promising technique because it is non-invasive and relatively low-cost, and somatic cells can be used instead of embryonic cells. More importantly, stem cells derived from urine did not form any tumours, and the use of autologous cells reduces the chances of rejection [ 147 ].
Use of graphene in stem cell therapy
Over recent years, graphene and its derivatives have been increasingly used as scaffold materials to mediate stem cell growth and differentiation [ 148 ]. Both graphene and graphene oxide (GO) represent high in-plane stiffness [ 149 ]. Because graphene has carbon and aromatic network, it works either covalently or non-covalently with biomolecules; in addition to its superior mechanical properties, graphene offers versatile chemistry. Graphene exhibits biocompatibility with cells and their proper adhesion. It also tested positively for enhancing the proliferation or differentiation of stem cells [ 148 ]. After positive experiments, graphene revealed great potential as a scaffold and guide for specific lineages of stem cell differentiation [ 150 ]. Graphene has been successfully used in the transplantation of hMSCs and their guided differentiation to specific cells. The acceleration skills of graphene differentiation and division were also investigated. It was discovered that graphene can serve as a platform with increased adhesion for both growth factors and differentiation chemicals. It was also discovered that π-π binding was responsible for increased adhesion and played a crucial role in inducing hMSC differentiation [ 150 ].
Therapeutic potential of extracellular vesicle-based therapies
Extracellular vesicles (EVs) can be released by virtually every cell of an organism, including stem cells [ 151 ], and are involved in intercellular communication through the delivery of their mRNAs, lipids, and proteins. As Oh et al. [ 152 ] prove, stem cells, together with their paracrine factors—exosomes—can become potential therapeutics in the treatment of, e.g. skin ageing. Exosomes are small membrane vesicles secreted by most cells (30–120 nm in diameter) [ 153 ]. When endosomes fuse with the plasma membrane, they become exosomes that have messenger RNAs (mRNAs) and microRNAs (miRNAs), some classes of non-coding RNAs (IncRNAs) and several proteins that originate from the host cell [ 154 ]. IncRNAs can bind to specific loci and create epigenetic regulators, which leads to the formation of epigenetic modifications in recipient cells. Because of this feature, exosomes are believed to be implicated in cell-to-cell communication and the progression of diseases such as cancer [ 155 ]. Recently, many studies have also shown the therapeutic use of exosomes derived from stem cells, e.g. skin damage and renal or lung injuries [ 156 ].
In skin ageing, the most important factor is exposure to UV light, called “photoageing” [ 157 ], which causes extrinsic skin damage, characterized by dryness, roughness, irregular pigmentation, lesions, and skin cancers. In intrinsic skin ageing, on the other hand, the loss of elasticity is a characteristic feature. The skin dermis consists of fibroblasts, which are responsible for the synthesis of crucial skin elements, such as procollagen or elastic fibres. These elements form either basic framework extracellular matrix constituents of the skin dermis or play a major role in tissue elasticity. Fibroblast efficiency and abundance decrease with ageing [ 158 ]. Stem cells can promote the proliferation of dermal fibroblasts by secreting cytokines such as platelet-derived growth factor (PDGF), transforming growth factor β (TGF-β), and basic fibroblast growth factor. Huh et al. [ 159 ] mentioned that a medium of human amniotic fluid-derived stem cells (hAFSC) positively affected skin regeneration after longwave UV-induced (UVA, 315–400 nm) photoageing by increasing the proliferation and migration of dermal fibroblasts. It was discovered that, in addition to the induction of fibroblast physiology, hAFSC transplantation also improved diseases in cases of renal pathology, various cancers, or stroke [ 160 , 161 ].
Oh [ 162 ] also presented another option for the treatment of skin wounds, either caused by physical damage or due to diabetic ulcers. Induced pluripotent stem cell-conditioned medium (iPSC-CM) without any animal-derived components induced dermal fibroblast proliferation and migration.
Natural cutaneous wound healing is divided into three steps: haemostasis/inflammation, proliferation, and remodelling. During the crucial step of proliferation, fibroblasts migrate and increase in number, indicating that it is a critical step in skin repair, and factors such as iPSC-CM that impact it can improve the whole cutaneous wound healing process. Paracrine actions performed by iPSCs are also important for this therapeutic effect [ 163 ]. These actions result in the secretion of cytokines such as TGF-β, interleukin (IL)-6, IL-8, monocyte chemotactic protein-1 (MCP-1), vascular endothelial growth factor (VEGF), platelet-derived growth factor-AA (PDGF-AA), and basic fibroblast growth factor (bFGF). Bae et al. [ 164 ] mentioned that TGF-β induced the migration of keratinocytes. It was also demonstrated that iPSC factors can enhance skin wound healing in vivo and in vitro when Zhou et al. [ 165 ] enhanced wound healing, even after carbon dioxide laser resurfacing in an in vivo study.
Peng et al. [ 166 ] investigated the effects of EVs derived from hESCs on in vitro cultured retinal glial, progenitor Müller cells, which are known to differentiate into retinal neurons. EVs appear heterogeneous in size and can be internalized by cultured Müller cells, and their proteins are involved in the induction and maintenance of stem cell pluripotency. These stem cell-derived vesicles were responsible for the neuronal trans-differentiation of cultured Müller cells exposed to them. However, the research article points out that the procedure was accomplished only on in vitro acquired retina.
Challenges concerning stem cell therapy
Although stem cells appear to be an ideal solution for medicine, there are still many obstacles that need to be overcome in the future. One of the first problems is ethical concern.
The most common pluripotent stem cells are ESCs. Therapies concerning their use at the beginning were, and still are, the source of ethical conflicts. The reason behind it started when, in 1998, scientists discovered the possibility of removing ESCs from human embryos. Stem cell therapy appeared to be very effective in treating many, even previously incurable, diseases. The problem was that when scientists isolated ESCs in the lab, the embryo, which had potential for becoming a human, was destroyed (Fig. 8 ). Because of this, scientists, seeing a large potential in this treatment method, focused their efforts on making it possible to isolate stem cells without endangering their source—the embryo.
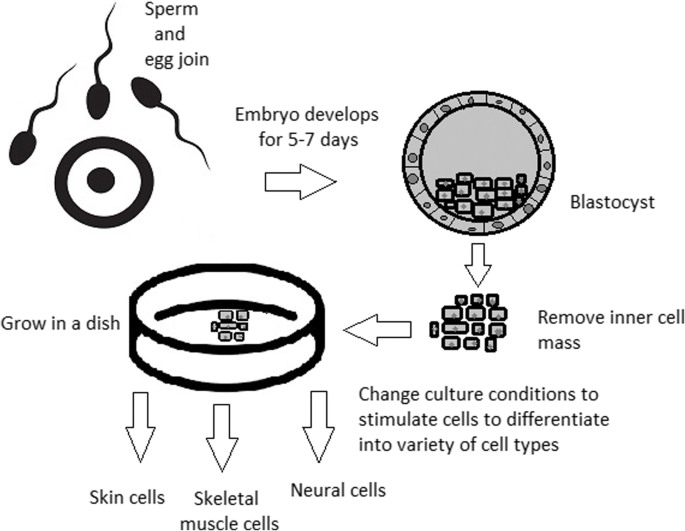
Use of inner cell mass pluripotent stem cells and their stimulation to differentiate into desired cell types
For now, while hESCs still remain an ethically debatable source of cells, they are potentially powerful tools to be used for therapeutic applications of tissue regeneration. Because of the complexity of stem cell control systems, there is still much to be learned through observations in vitro. For stem cells to become a popular and widely accessible procedure, tumour risk must be assessed. The second problem is to achieve successful immunological tolerance between stem cells and the patient’s body. For now, one of the best ideas is to use the patient’s own cells and devolve them into their pluripotent stage of development.
New cells need to have the ability to fully replace lost or malfunctioning natural cells. Additionally, there is a concern about the possibility of obtaining stem cells without the risk of morbidity or pain for either the patient or the donor. Uncontrolled proliferation and differentiation of cells after implementation must also be assessed before its use in a wide variety of regenerative procedures on living patients [ 167 ].
One of the arguments that limit the use of iPSCs is their infamous role in tumourigenicity. There is a risk that the expression of oncogenes may increase when cells are being reprogrammed. In 2008, a technique was discovered that allowed scientists to remove oncogenes after a cell achieved pluripotency, although it is not efficient yet and takes a longer amount of time. The process of reprogramming may be enhanced by deletion of the tumour suppressor gene p53, but this gene also acts as a key regulator of cancer, which makes it impossible to remove in order to avoid more mutations in the reprogrammed cell. The low efficiency of the process is another problem, which is progressively becoming reduced with each year. At first, the rate of somatic cell reprogramming in Yamanaka’s study was up to 0.1%. The use of transcription factors creates a risk of genomic insertion and further mutation of the target cell genome. For now, the only ethically acceptable operation is an injection of hESCs into mouse embryos in the case of pluripotency evaluation [ 168 ].
Stem cell obstacles in the future
Pioneering scientific and medical advances always have to be carefully policed in order to make sure they are both ethical and safe. Because stem cell therapy already has a large impact on many aspects of life, it should not be treated differently.
Currently, there are several challenges concerning stem cells. First, the most important one is about fully understanding the mechanism by which stem cells function first in animal models. This step cannot be avoided. For the widespread, global acceptance of the procedure, fear of the unknown is the greatest challenge to overcome.
The efficiency of stem cell-directed differentiation must be improved to make stem cells more reliable and trustworthy for a regular patient. The scale of the procedure is another challenge. Future stem cell therapies may be a significant obstacle. Transplanting new, fully functional organs made by stem cell therapy would require the creation of millions of working and biologically accurate cooperating cells. Bringing such complicated procedures into general, widespread regenerative medicine will require interdisciplinary and international collaboration.
The identification and proper isolation of stem cells from a patient’s tissues is another challenge. Immunological rejection is a major barrier to successful stem cell transplantation. With certain types of stem cells and procedures, the immune system may recognize transplanted cells as foreign bodies, triggering an immune reaction resulting in transplant or cell rejection.
One of the ideas that can make stem cells a “failsafe” is about implementing a self-destruct option if they become dangerous. Further development and versatility of stem cells may cause reduction of treatment costs for people suffering from currently incurable diseases. When facing certain organ failure, instead of undergoing extraordinarily expensive drug treatment, the patient would be able to utilize stem cell therapy. The effect of a successful operation would be immediate, and the patient would avoid chronic pharmacological treatment and its inevitable side effects.
Although these challenges facing stem cell science can be overwhelming, the field is making great advances each day. Stem cell therapy is already available for treating several diseases and conditions. Their impact on future medicine appears to be significant.
After several decades of experiments, stem cell therapy is becoming a magnificent game changer for medicine. With each experiment, the capabilities of stem cells are growing, although there are still many obstacles to overcome. Regardless, the influence of stem cells in regenerative medicine and transplantology is immense. Currently, untreatable neurodegenerative diseases have the possibility of becoming treatable with stem cell therapy. Induced pluripotency enables the use of a patient’s own cells. Tissue banks are becoming increasingly popular, as they gather cells that are the source of regenerative medicine in a struggle against present and future diseases. With stem cell therapy and all its regenerative benefits, we are better able to prolong human life than at any time in history.
Abbreviations
Basic fibroblast growth factor
Bone morphogenic proteins
Dental follicle stem cells
Dental pulp stem cells
Embryonic bodies
Embryonic stem cells
Fibroblast growth factors
Good Manufacturing Practice
Graphene oxide
Human amniotic fluid-derived stem cells
Human embryonic stem cells
Human foreskin fibroblasts
Inner cell mass
Non-coding RNA
Induced pluripotent stem cells
In vitro fertilization
Knockout serum replacement
Leukaemia inhibitory factor
Monocyte chemotactic protein-1
Fibroblasts
Messenger RNA
Mesenchymal stem cells of dental pulp
Myogenic differentiation
Osteoarthritis
Octamer-binding transcription factor 3 and 4
Platelet-derived growth factor
Platelet-derived growth factor-AA
Periodontal ligament stem cells
Rho-associated protein kinase
Stem cells from apical papilla
Stem cells of human exfoliated deciduous teeth
Synthetic Serum Substitute
Trophectoderm
Vascular endothelial growth factor
Transforming growth factors
Sukoyan MA, Vatolin SY, et al. Embryonic stem cells derived from morulae, inner cell mass, and blastocysts of mink: comparisons of their pluripotencies. Embryo Dev. 1993;36(2):148–58
Larijani B, Esfahani EN, Amini P, Nikbin B, Alimoghaddam K, Amiri S, Malekzadeh R, Yazdi NM, Ghodsi M, Dowlati Y, Sahraian MA, Ghavamzadeh A. Stem cell therapy in treatment of different diseases. Acta Medica Iranica. 2012:79–96 https://www.ncbi.nlm.nih.gov/pubmed/22359076 .
Sullivan S, Stacey GN, Akazawa C, et al. Quality guidelines for clinical-grade human induced pluripotent stem cell lines. Regenerative Med. 2018; https://doi.org/10.2217/rme-2018-0095 .
Amps K, Andrews PW, et al. Screening ethnically diverse human embryonic stem cells identifies a chromosome 20 minimal amplicon conferring growth advantage. Nat. Biotechnol. 2011; 29 (12):1121–44.
Google Scholar
Amit M, Itskovitz-Eldor J. Atlas of human pluripotent stem cells: derivation and culturing. New York: Humana Press; 2012.
Ludwig TE, Bergendahl V, Levenstein ME, Yu J, Probasco MD, Thomson JA. Feeder-independent culture of human embryonic stem cells. Nat Methods. 2006;3:637–46.
CAS PubMed Google Scholar
Kang MI. Transitional CpG methylation between promoters and retroelements of tissue-specific genes during human mesenchymal cell differentiation. J. Cell Biochem. 2007;102:224–39.
Vaes B, Craeye D, Pinxteren J. Quality control during manufacture of a stem cell therapeutic. BioProcess Int. 2012;10:50–5.
Bloushtain-Qimron N. Epigenetic patterns of embryonic and adult stem cells. Cell Cycle. 2009;8:809–17.
Brindley DA. Peak serum: implications of serum supply for cell therapy manufacturing. Regenerative Medicine. 2012;7:809–17.
Solter D, Knowles BB. Immunosurgery of mouse blastocyst. Proc Natl Acad Sci U S A. 1975;72:5099–102.
CAS PubMed PubMed Central Google Scholar
Hoepfl G, Gassmann M, Desbaillets I. Differentiating embryonic stem cells into embryoid bodies. Methods Mole Biol. 2004;254:79–98 https://doi.org/10.1385/1-59259-741-6:079 .
Lim WF, Inoue-Yokoo T, Tan KS, Lai MI, Sugiyama D. Hematopoietic cell differentiation from embryonic and induced pluripotent stem cells. Stem Cell Res Ther. 2013;4(3):71. https://doi.org/10.1186/scrt222 .
Article CAS PubMed PubMed Central Google Scholar
Mohr JC, de Pablo JJ, Palecek SP. 3-D microwell culture of human embryonic stem cells. Biomaterials. 2006;27(36):6032–42. https://doi.org/10.1016/j.biomaterials.2006.07.012 .
Article CAS PubMed Google Scholar
Doetschman TC, Eistetter H, Katz M, Schmidt W, Kemler R. The in vitro development of blastocyst-derived embryonic stem cell lines: formation of the visceral yolk sac, blood islands, and myocardium. J Embryol Exp Morphol. 1985;87:27–45.
Kurosawa HY. Methods for inducing embryoid body formation: in vitro differentiation system of embryonic stem cells. J Biosci Bioeng. 2007;103:389–98.
Heins N, Englund MC, Sjoblom C, Dahl U, Tonning A, Bergh C, Lindahl A, Hanson C, Semb H. Derivation, characterization, and differentiation of human embryonic stem cells. Stem Cells. 2004;22:367–76.
Rosowski KA, Mertz AF, Norcross S, Dufresne ER, Horsley V. Edges of human embryonic stem cell colonies display distinct mechanical properties and differentiation potential. Sci Rep. 2015;5:Article number:14218.
PubMed Google Scholar
Chung Y, Klimanskaya I, Becker S, Li T, Maserati M, Lu SJ, Zdravkovic T, Ilic D, Genbacev O, Fisher S, Krtolica A, Lanza R. Human embryonic stem cell lines generated without embryo destruction. Cell Stem Cell. 2008;2:113–7.
Zhang X, Stojkovic P, Przyborski S, Cooke M, Armstrong L, Lako M, Stojkovic M. Derivation of human embryonic stem cells from developing and arrested embryos. Stem Cells. 2006;24:2669–76.
Beers J, Gulbranson DR, George N, Siniscalchi LI, Jones J, Thomson JA, Chen G. Passaging and colony expansion of human pluripotent stem cells by enzyme-free dissociation in chemically defined culture conditions. Nat Protoc. 2012;7:2029–40.
Ellerström C, Hyllner J, Strehl R. single cell enzymatic dissociation of human embryonic stem cells: a straightforward, robust, and standardized culture method. In: Turksen K, editor. Human embryonic stem cell protocols. Methods in molecular biology: Humana Press; 2009. p. 584.
Heng BC, Liu H, Ge Z, Cao T. Mechanical dissociation of human embryonic stem cell colonies by manual scraping after collagenase treatment is much more detrimental to cellular viability than is trypsinization with gentle pipetting. Biotechnol Appl Biochem. 2010;47(1):33–7.
Ellerstrom C, Strehl R, Noaksson K, Hyllner J, Semb H. Facilitated expansion of human embryonic stem cells by single-cell enzymatic dissociation. Stem Cells. 2007;25:1690–6.
Brimble SN, Zeng X, Weiler DA, Luo Y, Liu Y, Lyons IG, Freed WJ, Robins AJ, Rao MS, Schulz TC. Karyotypic stability, genotyping, differentiation, feeder-free maintenance, and gene expression sampling in three human embryonic stem cell lines deri. Stem Cells Dev. 2004;13:585–97.
Watanabe K, Ueno M, Kamiya D, Nishiyama A, Matsumura M, Wataya T, Takahashi JB, Nishikawa S, Nishikawa S, Muguruma K, Sasai Y. A ROCK inhibitor permits survival of dissociated human embryonic stem cells. Nat Biotechnol. 2007;25:681–6.
Nie Y, Walsh P, Clarke DL, Rowley JA, Fellner T. Scalable passaging of adherent human pluripotent stem cells. 2014. https://doi.org/10.1371/journal.pone.0088012 .
Thomson JA, Itskovitz-Eldor J, Shapiro SS, Waknitz MA, Swiergiel JJ, Marshall VS, Jones JM. Embryonic stem cell lines derived from human blastocysts. Science. 1998;282:1145–7.
Martin MJ, Muotri A, Gage F, Varki A. Human embryonic stem cellsexpress an immunogenic nonhuman sialic acid. Nat. Med. 2005;11:228–32.
Smith AG, Heath JK, Donaldson DD, Wong GG, Moreau J, Stahl M, Rogers D. Inhibition of pluripotential embryonic stem cell differentiation by purified polypeptides. Nature. 1988;336(6200):688–90. https://doi.org/10.1038/336688a0 .
Xu C, Inokuma MS, Denham J, Golds K, Kundu P, Gold JD, Carpenter MK. Feeder-free growth of undifferentiated human embryonic stem cells. Nature Biotechnol. 2001;19:971–4. https://doi.org/10.1038/nbt1001-971 .
Article CAS Google Scholar
Weathersbee PS, Pool TB, Ord T. Synthetic serum substitute (SSS): a globulin-enriched protein supplement for human embryo culture. J. Assist Reprod Genet. 1995;12:354–60.
Chen G, Gulbranson DR, Hou Z, Bolin JM, Ruotti V, Probasco MD, Smuga-Otto K, Howden SE, Diol NR, Propson NE, Wagner R, Lee GO, Antosiewicz-Bourget J, Teng JM, Thomson JA. Chemically defined conditions for human iPSC derivation and culture. Nat. Methods. 2011;8:424–9.
Sommer CA, Mostoslavsky G. Experimental approaches for the generation of induced pluripotent stem cells. Stem Cell Res Ther. 2010;1:26.
PubMed PubMed Central Google Scholar
Takahashi K, Yamanaka S. Induced pluripotent stem cells in medicine and biology. Development. 2013;140(12):2457–61 https://doi.org/10.1242/dev.092551 .
Shi D, Lu F, Wei Y, et al. Buffalos ( Bubalus bubalis ) cloned by nuclear transfer of somatic cells. Biol. Reprod. 2007;77:285–91. https://doi.org/10.1095/biolreprod.107.060210 .
Gurdon JB. The developmental capacity of nuclei taken from intestinal epithelium cells of feeding tadpoles. Development. 1962;10:622–40 http://dev.biologists.org/content/10/4/622 .
CAS Google Scholar
Kain K. The birth of cloning: an interview with John Gurdon. Dis Model Mech. 2009;2(1–2):9–10. https://doi.org/10.1242/dmm.002014 .
Article PubMed Central Google Scholar
Davis RL, Weintraub H, Lassar AB. Expression of a single transfected cDNA converts fibroblasts to myoblasts. Cell. 1987;24(51(6)):987–1000.
Quinlan AR, Boland MJ, Leibowitz ML, et al. Genome sequencing of mouse induced pluripotent stem cells reveals retroelement stability and infrequent DNA rearrangement during reprogramming. Cell Stem Cell. 2011;9(4):366–73.
Maherali N, Sridharan R, Xie W, Utika LJ, Eminli S, Arnold K, Stadtfeld M, Yachechko R, Tchieu J, Jaenisch R, Plath K, Hochedlinger K. Directly reprogrammed fibroblasts show global epigenetic remodeling and widespread tissue contribution. Cell Stem Cell. 2007;1:55–70.
Ohi Y, Qin H, Hong C, Blouin L, Polo JM, Guo T, Qi Z, Downey SL, Manos PD, Rossi DJ, Yu J, Hebrok M, Hochedlinger K, Costello JF, Song JS, Ramalho-Santos M. Incomplete DNA methylation underlines a transcriptional memory of somatic cells in human IPS cells. Nat Cell Biol. 2011;13:541–9.
Zhou Q, Brown J, Kanarek A, Rajagopal J, Melton DA. In vivo reprogramming of adult pancreatic exocrine cells to beta-cells. Nature. 2008;455:627–32 https://doi.org/10.1038/nature07314 .
Hilfiker A, Kasper C, Hass R, Haverich A. Mesenchymal stem cells and progenitor cells in connective tissue engineering and regenerative medicine: is there a future for transplantation? Langenbecks Arch Surg. 2011;396:489–97.
Zhang Wendy, Y., de Almeida Patricia, E., and Wu Joseph, C. Teratoma formation: a tool for monitoring pluripotency in stem cell research. StemBook, ed. The Stem Cell Research Community . June 12, 2012. https://doi.org/10.3824/stembook.1.53.1 .
Narsinh KH, Sun N, Sanchez-Freire V, et al. Single cell transcriptional profiling reveals heterogeneity of human induced pluripotent stem cells. J Clin Invest. 2011;121(3):1217–21.
Gertow K, Przyborski S, Loring JF, Auerbach JM, Epifano O, Otonkoski T, Damjanov I, AhrlundRichter L. Isolation of human embryonic stem cell-derived teratomas for the assessment of pluripotency. Curr Protoc Stem Cell Biol . 2007, Chapter 1, Unit 1B 4. 3: 1B.4.1-1B.4.29.
Cooke MJ, Stojkovic M, Przyborski SA. Growth of teratomas derived from human pluripotent stem cells is influenced by the graft site. Stem Cells Dev. 2006;15(2):254–9.
Przyborski SA. Differentiation of human embryonic stem cells after transplantation in immune-deficient mice. Stem Cells. 2005;23:1242–50.
Tannenbaum SE, Turetsky TT, Singer O, Aizenman E, Kirshberg S, Ilouz N, Gil Y, Berman-Zaken Y, Perlman TS, Geva N, Levy O, Arbell D, Simon A, Ben-Meir A, Shufaro Y, Laufer N, Reubinoff BE. Derivation of xeno-free and GMP-grade human embryonic stem cells- platforms for future clinical applications. PLoS One. 2012;7:e35325.
Cohen DE, Melton D. Turning straw into gold: directing cell fate for regenerative medicine. Nat Rev Genet. 2011;12:243–52.
Hwang NS, Varghese S, Elisseeff J. Controlled differentiation of stem cells. Adv Drug Deliv Rev. 2007;60(2):199–214. https://doi.org/10.1016/j.addr.2007.08.036 .
Turner N, Grose R. Fibroblast growth factor signalling: from development to cancer. Nat Rev Cancer. 2010;10:116–29.
Rao TP, Kuhl M. An updated overview on Wnt signaling pathways: a prelude for more. Circ Res. 2010;106:1798–806.
Moustakas A, Heldin CH. The regulation of TGFbeta signal transduction. Development. 2009;136:3699–714.
Efthymiou AG, Chen G, Rao M, Chen G, Boehm M. Self-renewal and cell lineage differentiation strategies in human embryonic stem cells and induced pluripotent stem cells. Expert Opin Biol Ther. 2014;14:1333–44.
Yang L, Soonpaa MH, Adler ED, Roepke TK, Kattman SJ, Kennedy M, Henckaerts E, Bonham K, Abbott GW, Linden RM, Field LJ, Keller GM. Human cardiovascular progenitor cells develop from a KDRþembryonic-stem-cell-derived population. Nature. 2008;453:524–8.
Kroon E, Martinson LA, Kadoya K, Bang AG, Kelly OG, Eliazer S, Young H, Richardson M, Smart NG, Cunningham J, Agulnick AD, D’amour KA, Carpenter MK, Baetge EE. Pancreatic endoderm derived from human embryonic stem cells generates glucose-responsive insulin-secreting cells in vivo. Nat Biotechnol. 2008;26(4):443–52. https://doi.org/10.1038/nbt1393 .
Vallier L, Reynolds D, Pedersen RA. Nodal inhibits differentiation of human embryonic stem cells along the neuroectodermal default pathway. Dev Biol. 2004;275:403–21.
Burridge PW, Zambidis ET. Highly efficient directed differentiation of human induced pluripotent stem cells into cardiomyocytes. Methods Mol Biol. 2013;997:149–61.
Cai J, Zhao Y, Liu Y, Ye F, Song Z, Qin H, Meng S, Chen Y, Zhou R, Song X, Guo Y, Ding M, Deng H. Directed differentiation of human embryonic stem cells into functional hepatic cells. Hepatology. 2007;45:1229–39.
Takasato M, Er PX, Becroft M, Vanslambrouck JM, Stanley EG, Elefanty AG, Little MH. Directing human embryonic stem cell differentiation towards a renal lineage generates a selforganizing kidney. Nat Cell Biol. 2014;16:118–26.
Huang SX, Islam MN, O’Neill J, Hu Z, Yang YG, Chen YW, Mumau M, Green MD, VunjakNovakovic G, Bhattacharya J, Snoeck HW. Efficient generation of lung and airway epithelial cells from human pluripotent stem cells. Nat Biotechnol. 2014;32:84–91.
Kadzik RS, Morrisey EE. Directing lung endoderm differentiation in pluripotent stem cells. Cell Stem Cell. 2012;10:355–61.
Wichterle H, Lieberam I, Porter JA, Jessell TM. Directed differentiation of embryonic stem cells into motor neurons. Cell. 2002;110:385–97.
Spence JR, Mayhew CN, Rankin SA, Kuhar MF, Vallance JE, Tolle K, Hoskins EE, Kalinichenko VV, Wells SI, Zorn AM, Shroyer NF, Wells JM. Directed differentiation of human pluripotent stem cells into intestinal tissue in vitro. Nature. 2011;470:105–9.
Oldershaw RA, Baxter MA, Lowe ET, Bates N, Grady LM, Soncin F, Brison DR, Hardingham TE, Kimber SJ. Directed differentiation of human embryonic stem cells toward chondrocytes. Nat Biotechnol. 2010;28:1187–94.
Jun Cai, Ann DeLaForest, Joseph Fisher, Amanda Urick, Thomas Wagner, Kirk Twaroski, Max Cayo, Masato Nagaoka, Stephen A Duncan. Protocol for directed differentiation of human pluripotent stem cells toward a hepatocyte fate. 2012. DOI: https://doi.org/10.3824/stembook.1.52.1 .
Frank-Kamenetsky M, Zhang XM, Bottega S, Guicherit O, Wichterle H, Dudek H, Bumcrot D, Wang FY, Jones S, Shulok J, Rubin LL, Porter JA. Small-molecule modulators of hedgehog signaling: identification and characterization of smoothened agonists and antagonists. J Biol. 2002;1:10.
Oshima K, Shin K, Diensthuber M, Peng AW, Ricci AJ, Heller S. Mechanosensitive hair celllike cells from embryonic and induced pluripotent stem cells. Cell. 2010;141:704–16.
Osakada F, Jin ZB, Hirami Y, Ikeda H, Danjyo T, Watanabe K, Sasai Y, Takahashi M. In vitro differentiation of retinal cells from human pluripotent stem cells by small-molecule induction. J Cell Sci. 2009;122:3169–79.
Kshitiz PJ, Kim P, Helen W, Engler AJ, Levchenko A, Kim DH. Control of stem cell fate and function by engineering physical microenvironments. Intergr Biol (Camb). 2012;4:1008–18.
Amps K, Andrews PW, Anyfantis G, Armstrong L, Avery S, Baharvand H, Baker J, Baker D, Munoz MB, Beil S, Benvenisty N, Ben-Yosef D, Biancotti JC, Bosman A, Brena RM, Brison D, Caisander G, Camarasa MV, Chen J, ChiaoE CYM, Choo AB, Collins D, et al. Screening ethnically diverse human embryonic stem cells identifies a chromosome 20 minimal amplicon conferring growth advantage. Nat Biotechnol. 2011;29:1132–44.
Nukaya D, Minami K, Hoshikawa R, Yokoi N, Seino S. Preferential gene expression and epigenetic memory of induced pluripotent stem cells derived from mouse pancreas. Genes Cells. 2015;20:367–81.
Murdoch A, Braude P, Courtney A, Brison D, Hunt C, Lawford-Davies J, Moore H, Stacey G, Sethe S, Procurement Working Group Of National Clinical H, E. S. C. F, National Clinical H, E. S. C. F. The procurement of cells for the derivation of human embryonic stem cell lines for therapeutic use: recommendations for good practice. Stem Cell Rev. 2012;8:91–9.
Hewitson H, Wood V, Kadeva N, Cornwell G, Codognotto S, Stephenson E, Ilic D. Generation of KCL035 research grade human embryonic stem cell line carrying a mutation in HBB gene. Stem Cell Res. 2016;16:210–2.
Daley GQ, Hyun I, Apperley JF, Barker RA, Benvenisty N, Bredenoord AL, Breuer CK, Caulfield T, Cedars MI, Frey-Vasconcells J, Heslop HE, Jin Y, Lee RT, Mccabe C, Munsie M, Murry CE, Piantadosi S, Rao M, Rooke HM, Sipp D, Studer L, Sugarman J, et al. Setting global standards for stem cell research and clinical translation: the 2016 ISSCR guidelines. Stem Cell Rep. 2016;6:787–97.
Takahashi K, Yamanaka S. Induction of pluripotent stem cells from mouse embryonic and adult fibroblast cultures by defined factors. Cell. 2006;126(4):663–76. https://doi.org/10.1016/j.cell.2006.07.024 .
Loh YH, Wu Q, Chew JL, Vega VB, Zhang W, Chen X, Bourque G, George J, Leong B, Liu J, et al. The Oct4 and Nanog transcription network regulates pluripotency in mouse embryonic stem cells. Nat Genet. 2006;38:431–40.
Menasche P, Vanneaux V, Hagege A, Bel A, Cholley B, Cacciapuoti I, Parouchev A, Benhamouda N, Tachdjian G, Tosca L, Trouvin JH, Fabreguettes JR, Bellamy V, Guillemain R, SuberbielleBoissel C, Tartour E, Desnos M, Larghero J. Human embryonic stem cell-derived cardiac progenitors for severe heart failure treatment: first clinical case report. Eur Heart J. 2015;36:2011–7.
Schwartz SD, Regillo CD, Lam BL, Eliott D, Rosenfeld PJ, Gregori NZ, Hubschman JP, Davis JL, Heilwell G, Spirn M, Maguire J, Gay R, Bateman J, Ostrick RM, Morris D, Vincent M, Anglade E, Del Priore LV, Lanza R. Human embryonic stem cell-derived retinal pigment epithelium in patients with age-related macular degeneration and Stargardt’s macular dystrophy: follow-up of two open-label phase 1/2 studies. Lancet. 2015;385:509–16.
Ilic D, Ogilvie C. Concise review: human embryonic stem cells-what have we done? What are we doing? Where are we going? Stem Cells. 2017;35:17–25.
Rocha V, et al. Clinical use of umbilical cord blood hematopoietic stem cells. Biol Blood Marrow Transplant. 2006;12(1):34–4.
Longo UG, Ronga M, Maffulli N. Sports Med Arthrosc 17:112–126. Achilles tendinopathy. Sports Med Arthrosc. 2009;17:112–26.
Tempfer H, Lehner C, Grütz M, Gehwolf R, Traweger A. Biological augmentation for tendon repair: lessons to be learned from development, disease, and tendon stem cell research. In: Gimble J, Marolt D, Oreffo R, Redl H, Wolbank S, editors. Cell engineering and regeneration. Reference Series in Biomedical Engineering. Cham: Springer; 2017.
Goldring MB, Goldring SR. Osteoarthritis. J Cell Physiol. 2007;213:626–34.
Widuchowski W, Widuchowski J, Trzaska T. Articular cartilage defects: study of 25,124 knee arthroscopies. Knee. 2007;14:177–82.
Li R, Lin Q-X, Liang X-Z, Liu G-B, et al. Stem cell therapy for treating osteonecrosis of the femoral head: from clinical applications to related basic research. Stem Cell Res Therapy. 2018;9:291 https://doi.org/10.1186/s13287-018-1018-7 .
Gangji V, De Maertelaer V, Hauzeur JP. Autologous bone marrow cell implantation in the treatment of non-traumatic osteonecrosis of the femoral head: five year follow-up of a prospective controlled study. Bone. 2011;49(5):1005–9.
Zhao D, Cui D, Wang B, Tian F, Guo L, Yang L, et al. Treatment of early stage osteonecrosis of the femoral head with autologous implantation of bone marrow-derived and cultured mesenchymal stem cells. Bone. 2012;50(1):325–30.
Sen RK, Tripathy SK, Aggarwal S, Marwaha N, Sharma RR, Khandelwal N. Early results of core decompression and autologous bone marrow mononuclear cells instillation in femoral head osteonecrosis: a randomized control study. J Arthroplast. 2012;27(5):679–86.
Lapasset L, Milhavet O, Prieur A, Besnard E, Babled A, Aït-Hamou N, Leschik J, Pellestor F, Ramirez JM, De Vos J, Lehmann S, Lemaitre JM. Rejuvenating senescent and centenarian human cells by reprogramming through the pluripotent state. Genes Dev. 2011;25:2248–53.
Sahin E, Depinho RA. Linking functional decline of telomeres, mitochondria and stem cells during ageing. Nature. 2010;464:520–8.
Petkovich DA, Podolskiy DI, Lobanov AV, Lee SG, Miller RA, Gladyshev VN. Using DNA methylation profiling to evaluate biological age and longevity interventions. Cell Metab. 2017;25:954–60 https://doi.org/10.1016/j.cmet.2017.03.016 .
Gerontology, Rejuvenation by cell reprogramming: a new horizon in. Rodolfo G. Goya, Marianne Lehmann, Priscila Chiavellini, Martina Canatelli-Mallat, Claudia B. Hereñú and Oscar A. Brown. Stem Cell Res Therapy . 2018, 9:349. https://doi.org/10.1186/s13287-018-1075-y .
Ocampo A, Reddy P, Martinez-Redondo P, Platero-Luengo A, Hatanaka F, Hishida T, Li M, Lam D, Kurita M, Beyret E, Araoka T, Vazquez-Ferrer E, Donoso D, Roman JLXJ, Rodriguez-Esteban C, Nuñez G, Nuñez Delicado E, Campistol JM, Guillen I, Guillen P, Izpisua. In vivo amelioration of age-associated hallmarks by partial reprogramming. Cell. 2016;167:1719–33.
de Lázaro I, Cossu G, Kostarelos K. Transient transcription factor (OSKM) expression is key towards clinical translation of in vivo cell reprogramming. EMBO Mol Med. 2017;9:733–6.
Sun S, Li ZQ, Glencer P, Cai BC, Zhang XM, Yang J, Li XR. Bringing the age-related macular degeneration high-risk allele age-related maculopathy susceptibility 2 into focus with stem cell technology. Stem Cell Res Ther. 2017;8:135 https://doi.org/10.1186/s13287-017-0584-4 .
Liu J. Induced pluripotent stem cell-derived neural stem cells: new hope for stroke? Stem Cell Res Ther. 2013;4:115 https://doi.org/10.1186/scrt326 .
Shahjalal HM, Dayem AA, Lim KM, Jeon TI, Cho SG. Generation of pancreatic β cells for treatment of diabetes: advances and challenges. Stem Cell ResTher. 2018;9:355 https://doi.org/10.1186/s13287-018-1099-3 .
Kroon E, Martinson LA, et al. Pancreatic endoderm derived from human embryonic stem cells generates glucose-responsive insulin-secreting cells in vivo. Nat Biotechnol. 2008;26;443–52.
Arora V, Pooja A, Munshi AK. Banking stem cells from human exfoliated deciduous teeth. J Clin Pediatr Dent. 2009;33(4):289–94.
Mao JJ. Stem cells and the future of dental care. New York State Dental J. 2008;74(2):21–4.
Reznick, Jay B. Continuing education: stem cells: emerging medical and dental therapies for the dental Professional. Dentaltown Magazine . 2008, pp. 42–53.
Arthur A, Rychkov G, Shi S, Koblar SA, Gronthos S. Adult human dental pulp stem cells differentiate toward functionally active neurons under appropriate environmental cues. Stem Cells. 2008;26(7):1787–95.
Cordeiro MM, Dong Z, Kaneko T, Zhang Z, Miyazawa M, Shi S, Smith A. Dental pulp tissue engineering with stem cells from exfoliated. J Endod. 2008;34(8):962–9.
Hayashi K, Ohta H, Kurimoto K, Aramaki S, Saitou M. Reconstitution of the mouse germ cell specification pathway in culture by pluripotent stem cells. Cell. 2011;146(4):519–32. https://doi.org/10.1016/j.cell.2011.06.052 .
Sadri-Ardekani H, Atala A. Testicular tissue cryopreservation and spermatogonial stem cell transplantation to restore fertility: from bench to bedside. Stem Cell ResTher. 2014;5:68 https://doi.org/10.1186/scrt457 .
Zhang Q, Xu M, Yao X, Li T, Wang Q, Lai D. Human amniotic epithelial cells inhibit granulosa cell apoptosis induced by chemotherapy and restore the fertility. Stem Cell Res Ther. 2015;6:152 https://doi.org/10.1186/s13287-015-0148-4 .
Ma DK, Bonaguidi MA, Ming GL, Song H. Adult neural stem cells in the mammalian central nervous system. Cell Res. 2009;19:672–82. https://doi.org/10.1038/cr.2009.56 .
Dantuma E, Merchant S, Sugaya K. Stem cells for the treatment of neurodegenerative diseases. Stem Cell ResTher. 2010;1:37 https://doi.org/10.1186/scrt37 .
Wang Q, Matsumoto Y, Shindo T, Miyake K, Shindo A, Kawanishi M, Kawai N, Tamiya T, Nagao S. Neural stem cells transplantation in cortex in a mouse model of Alzheimer’s disease. J Med Invest. 2006;53:61–9. https://doi.org/10.2152/jmi.53.61 .
Article PubMed Google Scholar
Moghadam FH, Alaie H, Karbalaie K, Tanhaei S, Nasr Esfahani MH, Baharvand H. Transplantation of primed or unprimed mouse embryonic stem cell-derived neural precursor cells improves cognitive function in Alzheimerian rats. Differentiation. 2009;78:59–68. https://doi.org/10.1016/j.diff.2009.06.005 .
Byrne JA. Developing neural stem cell-based treatments for neurodegenerative diseases. Stem Cell ResTher. 2014;5:72. https://doi.org/10.1186/scrt461 .
Awe JP, Lee PC, Ramathal C, Vega-Crespo A, Durruthy-Durruthy J, Cooper A, Karumbayaram S, Lowry WE, Clark AT, Zack JA, Sebastiano V, Kohn DB, Pyle AD, Martin MG, Lipshutz GS, Phelps PE, Pera RA, Byrne JA. Generation and characterization of transgene-free human induced pluripotent stem cells and conversion to putative clinical-grade status. Stem Cell Res Ther. 2013;4:87. https://doi.org/10.1186/scrt246 .
Peng J, Zeng X. The role of induced pluripotent stem cells in regenerative medicine: neurodegenerative diseases. Stem Cell ResTher. 2011;2:32. https://doi.org/10.1186/scrt73 .
Wright BL, Barker RA. Established and emerging therapies for Huntington’s disease. 2007;7(6):579–87 https://www.ncbi.nlm.nih.gov/pubmed/17896994/579-87 .
Lin NH, Gronthos S, Bartold PM. Stem cells and periodontal regeneration. Aust Dent J. 2008;53:108–21.
Seo BM, Miura M, Gronthos S, Bartold PM, Batouli S, Brahim J, et al. Investigation of multipotent postnatal stem cells from human periodontal ligament. Lancet. 2004;364:149–55.
Ramseier CA, Abramson ZR, Jin Q, Giannobile WV. Gene therapeutics for periodontal regenerative medicine. Dent Clin North Am. 2006;50:245–63.
Shi S, Bartold PM, Miura M, Seo BM, Robey PG, Gronthos S. The efficacy of mesenchymal stem cells to regenerate and repair dental structures. OrthodCraniofac Res. 2005;8:191–9.
Iohara K, Nakashima M, Ito M, Ishikawa M, Nakasima A, Akamine A. Dentin regeneration by dental pulp stem cell therapy with recombinant human bone morphogenetic protein. J Dent Res. 2004;83:590–5.
Hu B, Unda F, Bopp-Kuchler S, Jimenez L, Wang XJ, Haikel Y, et al. Bone marrow cells can give rise to ameloblast-like cells. J Dent Res. 2006;85:416–21.
Liu Y, Liu W, Hu C, Xue Z, Wang G, Ding B, Luo H, Tang L, Kong X, Chen X, Liu N, Ding Y, Jin Y. MiR-17 modulates osteogenic differentiation through a coherent feed-forward loop in mesenchymal stem cells isolated from periodontal ligaments of patients with periodontitis. Stem Cells. 2011;29(11):1804–16. https://doi.org/10.1002/stem.728 .
Raspini G, Wolff J, Helminen M, Raspini G, Raspini M, Sándor GK. Dental stem cells harvested from third molars combined with bioactive glass can induce signs of bone formation in vitro. J Oral Maxillofac Res. 2018;9(1):e2. Published 2018 Mar 31. https://doi.org/10.5037/jomr.2018.9102 .
Christodoulou I, Goulielmaki M, Devetzi M, Panagiotidis M, Koliakos G, Zoumpourlis V. Mesenchymal stem cells in preclinical cancer cytotherapy: a systematic review. Stem Cell Res Ther. 2018;9(1;336). https://doi.org/10.1186/s13287-018-1078-8 .
Bansal R, Jain A. Current overview on dental stem cells applications in regenerative dentistry. J Nat Sci Biol Med. 2015;6(1):29–34. https://doi.org/10.4103/0976-9668.149074 .
Article PubMed PubMed Central Google Scholar
Edgar Ledesma-Martínez, Víctor Manuel Mendoza-Núñez, Edelmiro Santiago-Osorio. Mesenchymal stem cells derived from dental pulp: a review. Stem Cells Int . 2016, 4,709,572, p. doi: https://doi.org/10.1155/2016/4709572 ].
Grzech-Leśniak K. Making use of lasers in periodontal treatment: a new gold standard? Photomed Laser Surg. 2017;35(10):513–4.
Miura M, Gronthos S, Zhao M, Lu B, Fisher LW, Robey PG, Shi S. SHED: stem cells from human exfoliated deciduous teeth. Proc Natl Acad Sci U S A. 2003;100(10):5807–12. https://doi.org/10.1073/pnas.0937635100 .
Yasui T, Mabuchi Y, Toriumi H, Ebine T, Niibe K, Houlihan DD, Morikawa S, Onizawa K, Kawana H, Akazawa C, Suzuki N, Nakagawa T, Okano H, Matsuzaki Y. Purified human dental pulp stem cells promote osteogenic regeneration. J Dent Res. 2016;95(2):206–14. https://doi.org/10.1177/0022034515610748 .
Yamamoto A, Sakai K, Matsubara K, Kano F, Ueda M. Multifaceted neuro-regenerative activities of human dental pulp stem cells for functional recovery after spinal cord injury. Neurosci Res. 2014;78:16–20. https://doi.org/10.1016/j.neures.2013.10.010 .
d’Aquino R, De Rosa A, Lanza V, Tirino V, Laino L, Graziano A, Desiderio V, Laino G, Papaccio G. Human mandible bone defect repair by the grafting of dental pulp stem/progenitor cells and collagen sponge biocomplexes. Eur Cell Mater. 2009;12, PMID: 19908196:75–83.
Wang L, Shen H, Zheng W, Tang L, Yang Z, Gao Y, Yang Q, Wang C, Duan Y, Jin Y. Characterization of stem cells from alveolar periodontal ligament. Tissue Eng. Part A. 2011;17(7–8):1015–26. https://doi.org/10.1089/ten.tea.2010.0140 .
Iwata T, Yamato M, Zhang Z, Mukobata S, Washio K, Ando T, Feijen J, Okano T, Ishikawa I. Validation of human periodontal ligament-derived cells as a reliable source for cytotherapeutic use. J Clin Periodontol. 2010;37(12):1088–99. https://doi.org/10.1111/j.1600-051X.2010.01597.x .
Chen F-M, Gao L-N, Tian B-M, Zhang X-Y, Zhang Y-J, Dong G-Y, Lu H, et al. Treatment of periodontal intrabony defects using autologous periodontal ligament stem cells: a randomized clinical trial. Stem Cell Res Ther. 2016;7:33. https://doi.org/10.1186/s13287-016-0288-1 .
Bakopoulou A, Leyhausen G, Volk J, Tsiftsoglou A, Garefis P, Koidis P, Geurtsen W. Comparative analysis of in vitro osteo/odontogenic differentiation potential of human dental pulp stem cells (DPSCs) and stem cells from the apical papilla (SCAP). Arch Oral Biol. 2011;56(7):709–21. https://doi.org/10.1016/j.archoralbio.2010.12.008 .
Han C, Yang Z, Zhou W, Jin F, Song Y, Wang Y, Huo N, Chen L, Qian H, Hou R, Duan Y, Jin Y. Periapical follicle stem cell: a promising candidate for cementum/periodontal ligament regeneration and bio-root engineering. Stem Cells Dev. 2010;19(9):1405–15. https://doi.org/10.1089/scd.2009.0277 .
Luan X, Ito Y, Dangaria S, Diekwisch TG. Dental follicle progenitor cell heterogeneity in the developing mouse periodontium. Stem Cells Dev. 2006;15(4):595–608. https://doi.org/10.1089/scd.2006.15.595 .
Handa K, Saito M, Tsunoda A, Yamauchi M, Hattori S, Sato S, Toyoda M, Teranaka T, Narayanan AS. Progenitor cells from dental follicle are able to form cementum matrix in vivo. Connect Tissue Res. 2002;43(2–3):406–8 PMID: 12489190.
Guo W, Chen L, Gong K, Ding B, Duan Y, Jin Y. Heterogeneous dental follicle cells and the regeneration of complex periodontal tissues. Tissue Engineering. Part A. 2012;18(5–6):459–70 https://doi.org/10.1089/ten.tea.2011.0261 .
Bai, Yudi et al. Cementum- and periodontal ligament-like tissue formation by dental follicle cell sheets co-cultured with Hertwig’s epithelial root sheath cells. Bone. 2011, 48, Issue 6, pp. 1417–1426, https://doi.org/10.1016/j.bone.2011.02.016 .
Cordeiro MM, Dong Z, Kaneko T, Zhang Z, Miyazawa M, Shi S, et al. Dental pulp tissue engineering with stem cells from exfoliated deciduous teeth. 2008, 34, pp. 962–969.
Dobie K, Smith G, Sloan AJ, Smith AJ. Effects of alginate, hydrogels and TGF-beta 1 on human dental pulp repair in vitro. Connect Tissue Res 2. 2002;43:387–90.
Friedlander LT, Cullinan MP, Love RM. Dental stem cells and their potential role in apexogenesis and apexification. Int Endod J. 2009;42:955–62.
Cai J, Zhang Y, Liu P, Chen S, Wu X, Sun Y, Li A, Huang K, Luo R, Wang L, Liu Y, Zhou T, Wei S, Pan G, Pei D, Generation of tooth-like structures from integration-free human urine induced pluripotent stem cells. Cell Regen (Lond). July 30, 2013, 2(1), pp. 6, doi: https://doi.org/10.1186/2045-9769-2-6 .
Craig J. Taylor, Eleanor M. Bolton, and J. Andrew Bradley 2011 Aug 12 and https://doi.org/10.1098/rstb.2011.0030 ], 366(1575): 2312–2322. [doi:. Immunological considerations for embryonic and induced pluripotent stem cell banking,. Philos Trans R SocLond B Biol Sci. 2011, 366(1575), pp. 2312–2322, doi: https://doi.org/10.1098/rstb.2011.0030 .
T.R. Nayak, H. Andersen, V.S. Makam, C. Khaw, S. Bae, X.F. Xu, P.L.R. Ee, J.H. Ahn, B.H. Hong, G. Pastorin, B. Ozyilmaz, ACS Nano, 5 (6) (2011), pp. 4. Graphene for controlled and accelerated osteogenic differentiation of human mesenchymal stem cells,. ACS Nano. 2011, pp. 4670–4678.
Lee WC, Lim C, Shi H, Tang LAL, Wang Y, Lim CT, Loh KP. Origin of enhanced stem cell growth and differentiation on graphene and graphene oxide. ACS Nano. 2011;5(9):7334–41.
Kenry LWC, Loh KP, Lim CT. When stem cells meet graphene: opportunities and challenges in regenerative medicine. Biomaterials. 2018;155:236–50.
Yuan A, Farber EL, Rapoport AL, Tejada D, Deniskin R, Akhmedov NB, et al. Transfer of microRNAs by embryonic stem cell microvesicles. 2009. 2009, 4(3), p. https://doi.org/10.1371/journal.pone . 0004722.
Oh, Myeongsik, et al. Exosomes derived from human induced pluripotent stem cells ameliorate the aging of skin fibroblasts. Int. J. Mol. Sci. 2018, 19(6), p. 1715.
Ramirez MI. et al. Technical challenges of working with extracellular vesicles. Nanoscale. 2018;10:881–906.
Valadi H, et al. Exosome-mediated transfer of mRNAs and microRNAs is a novel mechanism of genetic exchange between cells. Nat. Cell Biol. 2007;9:654–9.
Mateescu B, et al. Obstacles and opportunities in the functional analysis of extracellular vesicle RNA—an ISEV position paper. J. Extracell. Vesicles. 2017;6(1). https://doi.org/10.1080/20013078.2017.1286095 .
Nawaz M, et al. Extracellular vesicles: evolving factors in stem cell biology. Stem Cells Int. 2016;2016:17. Article ID 1073140.
Helfrich, Y.R., Sachs, D.L. and Voorhees, J.J. Overview of skin aging and photoaging. Dermatol. Nurs. 20, pp. 177–183, https://www.ncbi.nlm.nih.gov/pubmed/18649702 .
Julia Tigges, Jean Krutmann, Ellen Fritsche, Judith Haendeler, Heiner Schaal, Jens W. Fischer, Faiza Kalfalah, Hans Reinke, Guido Reifenberger, Kai Stühler, Natascia Ventura, Sabrina Gundermann, Petra Boukamp, Fritz Boege. The hallmarks of fibroblast ageing, mechanisms of ageing and development, 138, 2014, Pages 26–44. 2014, 138, pp. 26–44, ISSN 0047–6374, https://doi.org/10.1016/j.mad.2014.03.004 .
Huh MI, Kim MS, Kim HK, et al. Effect of conditioned media collected from human amniotic fluid-derived stem cells (hAFSCs) on skin regeneration and photo-aging. Tissue Eng Regen Med. 2014;11:171 https://doi.org/10.1007/s13770-014-0412-1 .
Togel F, Hu Z, Weiss K, et al. Administered mesenchymal stem cells protect against ischemic acute renal failure through differentiation-independent mechanisms. Am J Physiol Renal Physiol. 2005;289:F31.
Liu J, Han G, Liu H, et al. Suppression of cholangiocarcinoma cell growth by human umbilical cord mesenchymal stem cells: a possible role of Wnt and Akt signaling. PLoS One. 2013;8:e62844.
Oh M, et al. Promotive effects of human induced pluripotent stem cell-conditioned medium on the proliferation and migration of dermal fibroblasts. Biotechnol. Bioprocess Eng. 2017;22:561–8.
Chen L, Tredget EE, Wu PY, Wu Y. Paracrine factors of mesenchymal stem cells recruit macrophages and endothelial lineage cells and enhance wound healing. PloS One. 2008;3:e1886.
Bae J-S, Lee S-H, Kim J-E, Choi J-Y, Park R-W, Park JY, Park H-S, Sohn Y-S, Lee D-S, Lee EB. βig-h3 supports keratinocyte adhesion, migration, and proliferation through α3β1 integrin. Biochem. Biophys. Res. Commun. 2002;294:940–8.
Zhou B-R, Xu Y, Guo S-L, Xu Y, Wang Y, Zhu F, Permatasari F, Wu D, Yin Z-Q, Luo D. The effect of conditioned media of adipose-derived stem cells on wound healing after ablative fractional carbon dioxide laser resurfacing. BioMed Res. Int. 2013;519:126.
Peng Y, Baulier E, Ke Y, Young A, Ahmedli NB, Schwartz SD, et al. Human embryonic stem cells extracellular vesicles and their effects on immortalized human retinal Müller cells. PLoS ONE. 2018, 13(3), p. https://doi.org/10.1371/journal.pone.019400 .
Harris MT, Butler DL, Boivin GP, Florer JB, Schantz EJ, Wenstrup RJ. Mesenchymal stem cells used for rabbit tendon repair can form ectopic bone and express alkaline phosphatase activity in constructs. J Orthop Res. 2004;22:998–1003.
Mascetti VL, Pedersen RA. Human-mouse chimerism validates human stem cell pluripotency. Cell Stem Cell. 2016;18:67–72.
Gandia C, Armiñan A, García-Verdugo JM, Lledó E, Ruiz A, Miñana MD, Sanchez-Torrijos J, Payá R, Mirabet V, Carbonell-Uberos F, Llop M, Montero JA, Sepúlveda P. Human dental pulp stem cells improve left ventricular function, induce angiogenesis, and reduce infarct size in rats with acute myocardial infarction. Stem Cells. 2007;26(3):638–45.
Perry BC, Zhou D, Wu X, Yang FC, Byers MA, Chu TM, Hockema JJ, Woods EJ, Goebel WS. Collection, cryopreservation, and characterization of human dental pulp-derived mesenchymal stem cells for banking and clinical use. Tissue Eng Part C Methods. 2008;14(2):149–56.
Garcia-Olmo D, Garcia-Arranz M, Herreros D, et al. A phase I clinical trial of the treatment of Crohn’s fistula by adipose mesenchymal stem cell transplantation. Dis Colon Rectum. 2005;48:1416–23.
de Mendonça CA, Bueno DF, Martins MT, Kerkis I, Kerkis A, Fanganiello RD, Cerruti H, Alonso N, Passos-Bueno MR. Reconstruction of large cranial defects in nonimmunosuppressed experimental design with human dental pulp stem cells. J Craniofac Surg. 2008;19(1):204–10.
Seo BM, Sonoyama W, Yamaza T, Coppe C, Kikuiri T, Akiyama K, Lee JS, Shi S. SHED repair critical-size calvarial defects in mice. Oral Dis. 2008;14(5):428–34.
Abbas, Diakonov I., Sharpe P. Neural crest origin of dental stem cells. Pan European Federation of the International Association for Dental Research (PEF IADR). 2008, Vols. Seq #96 - Oral Stem Cells.
Kerkis I, Ambrosio CE, Kerkis A, Martins DS, Gaiad TP, Morini AC, Vieira NM, Marina P, et al. Early transplantation of human immature dental pulp stem cells from baby teeth to golden retriever muscular dystrophy (GRMD) dogs. J Transl Med. 2008;6:35.
Xianrui Yang, Li Li, Li Xiao, Donghui Zhang. Recycle the dental fairy’s package: overview of dental pulp stem cells. Stem Cell Res Ther . 2018, 9, 1, 1. https://doi.org/10.1186/s13287-018-1094-8 .
Wang J, Wang X, Sun Z, Wang X, Yang H, Shi S, Wang S. Stem cells from human-exfoliated deciduous teeth can differentiate into dopaminergic neuron-like cells. Stem Cells Dev. 2010;19:1375–83.
Wang J, et al. The odontogenic differentiation of human dental pulp stem cells on nanofibrous poly (L-lactic acid) scaffolds in vitro and in vivo. Acta Biomater. 2010;6(10):3856–63.
Davies OG, Cooper PR, Shelton RM, Smith AJ, Scheven BA. A comparison of the in vitro mineralisation and dentinogenic potential of mesenchymal stem cells derived from adipose tissue, bone marrow and dental pulp. J Bone Miner Metab. 2015;33:371–82.
Huang GT-J, Shagramanova K, Chan SW. Formation of odontoblast-like cells from cultured human dental pulp cells on dentin in vitro. J Endod. 2006;32:1066–73.
Shi S, Robey PG, Gronthos S. Comparison of human dental pulp and bone marrow stromal stem cells by cDNA microarray analysis. Bone. 2001;29(6):532–9.
Gronthos S, Mankani M, Brahim J, Robey PG, Shi S. Postnatal human dental pulp stem cells (DPSCs) in vitro and in vivo. Proc Natl Acad Sci U S A. 2000;97:13625–30.
Nuti N, Corallo C, Chan BMF, Ferrari M, Gerami-Naini B. Multipotent differentiation of human dental pulp stem cells: a literature review. Stem Cell Rev Rep. 2016;12:511–23.
Ferro F, et al. Dental pulp stem cells differentiation reveals new insights in Oct4A dynamics. PloS One. 2012;7(7):e41774.
Conde MCM, Chisini LA, Grazioli G, Francia A, Carvalho RVd, Alcázar JCB, Tarquinio SBC, Demarco FF. Does cryopreservation affect the biological properties of stem cells from dental tissues? A systematic review. Braz Dent J. 2016;1210(6):633-40. https://doi.org/10.1590/0103-6440201600980 .
Papaccio G, Graziano A, d’Aquino R, Graziano MF, Pirozzi G, Menditti D, De Rosa A, Carinci F, Laino G. Long-term cryopreservation of dental pulp stem cells (SBP-DPSCs) and their differentiated osteoblasts: a cell source for tissue repair. J Cell Physiol. 2006;208:319–25.
Alge DL, Zhou D, Adams LL, et. al. Donor-matched comparison of dental pulp stem cells and bone marrow-derived mesenchymal stem cells in a rat model. J Tissue Eng Regen Med. 2010;4(1):73–81.
Jo Y-Y, Lee H-J, Kook S-Y, Choung H-W, Park J-Y, Chung J-H, Choung Y-H, Kim E-S, Yang H-C, Choung P-H. Isolation and characterization of postnatal stem cells from human dental tissues. Tissue Eng. 2007;13:767–73.
Gronthos S, Brahim J, Li W, Fisher LW, Cherman N, Boyde A, DenBesten P, Robey PG, Shi S. Stem cell properties of human dental pulp stem cells. J Dent Res. 2002;81:531–5.
Laino G, d’Aquino R, Graziano A, Lanza V, Carinci F, Naro F, Pirozzi G, Papaccio G. A new population of human adult dental pulp stem cells: a useful source of living autologous fibrous bone tissue (LAB). J Bone Miner Res. 2005;20:1394–402.
Zainal A, Shahrul H, et al. In vitro chondrogenesis transformation study of mouse dental pulp stem cells. Sci World J. 2012;2012:827149.
Wei X, et al. Expression of mineralization markers in dental pulp cells. J Endod. 2007;33(6):703–8.
Dai J, et al. The effect of co-culturing costal chondrocytes and dental pulp stem cells combined with exogenous FGF9 protein on chondrogenesis and ossification in engineered cartilage. Biomaterials. 2012;33(31):7699–711.
Vasandan AB, et al. Functional differences in mesenchymal stromal cells from human dental pulp and periodontal ligament. J Cell Mol Med. 2014;18(2):344–54.
Werle SB, et al. Carious deciduous teeth are a potential source for dental pulp stem cells. Clin Oral Investig. 2015;20:75–81.
Nemeth CL, et al. Enhanced chondrogenic differentiation of dental pulp stem cells using nanopatterned PEG-GelMA-HA hydrogels. Tissue Eng A. 2014;20(21–22):2817–29.
Paino F, Ricci G, De Rosa A, D’Aquino R, Laino L, Pirozzi G, et al. Ecto-mesenchymal stem cells from dental pulp are committed to differentiate into active melanocytes. Eur. Cell Mater. 2010;20:295–305.
Ferro F, Spelat R, Baheney CS. Dental pulp stem cell (DPSC) isolation, characterization, and differentiation. In: Kioussi C, editor. Stem cells and tissue repair. Methods in molecular biology (methods and protocols): Humana Press. 2014;1210.
Ishkitiev N, Yaegaki K, Imai T, Tanaka T, Nakahara T, Ishikawa H, Mitev V, Haapasalo M. High-purity hepatic lineage differentiated from dental pulp stem cells in serum-free medium. J Endod. 2012;38:475–80.
Download references
Acknowledgements
Not applicable.
This work is supported by Wrocław Medical University in Poland.
Availability of data and materials
Please contact author for data requests.
Author information
Authors and affiliations.
Department of Experimental Surgery and Biomaterials Research, Wroclaw Medical University, Bujwida 44, Wrocław, 50-345, Poland
Wojciech Zakrzewski, Maria Szymonowicz & Zbigniew Rybak
Department of Conservative Dentistry and Pedodontics, Krakowska 26, Wrocław, 50-425, Poland
Maciej Dobrzyński
You can also search for this author in PubMed Google Scholar
Contributions
WZ is the principal author and was responsible for the first draft of the manuscript. WZ and ZR were responsible for the concept of the review. MS, MD, and ZR were responsible for revising the article and for data acquisition. All authors read and approved the final manuscript.
Corresponding author
Correspondence to Wojciech Zakrzewski .
Ethics declarations
Ethics approval and consent to participate, consent for publication, competing interests.
The authors declare that they have no competing interests.
Publisher’s Note
Springer Nature remains neutral with regard to jurisdictional claims in published maps and institutional affiliations.
Rights and permissions
Open Access This article is distributed under the terms of the Creative Commons Attribution 4.0 International License ( http://creativecommons.org/licenses/by/4.0/ ), which permits unrestricted use, distribution, and reproduction in any medium, provided you give appropriate credit to the original author(s) and the source, provide a link to the Creative Commons license, and indicate if changes were made. The Creative Commons Public Domain Dedication waiver ( http://creativecommons.org/publicdomain/zero/1.0/ ) applies to the data made available in this article, unless otherwise stated.
Reprints and permissions
About this article
Cite this article.
Zakrzewski, W., Dobrzyński, M., Szymonowicz, M. et al. Stem cells: past, present, and future. Stem Cell Res Ther 10 , 68 (2019). https://doi.org/10.1186/s13287-019-1165-5
Download citation
Published : 26 February 2019
DOI : https://doi.org/10.1186/s13287-019-1165-5
Share this article
Anyone you share the following link with will be able to read this content:
Sorry, a shareable link is not currently available for this article.
Provided by the Springer Nature SharedIt content-sharing initiative
- Differentiation
- Pluripotency
- Induced pluripotent stem cell (iPSC)
- Stem cell derivation
- Growth media
- Tissue banks
- Tissue transplantation
Stem Cell Research & Therapy
ISSN: 1757-6512
- Submission enquiries: Access here and click Contact Us
- General enquiries: [email protected]
More From Forbes
Ai tools fuel rise of fake research papers on google scholar.
- Share to Facebook
- Share to Twitter
- Share to Linkedin
AI ethics are in question when scientific papers use AI without disclosing or AI is tapped to ... [+] produce completely fake research results.
There’s a quote frequently attributed to Mark Twain that goes, “A lie can travel halfway around the world before the truth can get on its boots.” Whether or not Twain truly said that, a reality in the age of AI is it’s becoming increasingly difficult to distinguish truth from fiction .
New evidence supporting that fact comes from a group of Swedish researchers that just issued its findings regarding a growing number of fake scientific papers published to Google Scholar . The study found that more than 130 submissions either used AI without proper disclosure or were entirely faked using AI tools.
Google Scholar Not So Scholarly?
The researchers decided to conduct a mini-scrape of the Google Scholar index looking for two commonly generated phrases that public AI tools such as ChatGPT or Claude provide as part of the answers produced in response to prompts. The two phrases are:
- "as of my last knowledge update"
- "I don\'t have access to real-time data"
If either or both of those obvious genAI phrases were found in one of the papers uploaded to Google Scholar the team flagged it, and looked it over for proper acknowledgement that an AI tool was used as part of that specific paper’s study methodology.
The search flagged 227 papers, of which 139 papers failed to cite, mention or reference any use of AI—despite its clear use. It’s worth noting that Google Scholar reportedly has more than 389 million records on its website and the researchers’ sample represents a miniscule 0.0000003573% of all published papers.
Google Warns Millions Of Android Users—Do Not Install These Apps
Ukrainian troops breached russian border defenses 20 miles west of the kursk salient—but didn’t get very far, ‘shock and awe’—china could be about to trigger a $1.4 trillion bitcoin and crypto price earthquake.
Regardless, researcher Kristofer Rolf Söderström from Lund University, Sweden explained in an email exchange why his team’s study to callout sham science was necessary.
“With this research, we wanted to address the issue by looking into how common this is, especially because Google Scholar is so easy to use and it is very widely used, even by ourselves, but actually it is not that well controlled,” Söderström wrote.
“Our motivation was that the depth of the issue could be mitigated by such an investigation, thus making an early contribution to highlight the growing concern of undeclared GPT-use in academic papers since this runs the risk of ill-will hacking of society’s evidence base. But really, just the possibility of this happening—even if it is quite uncommon—risks further undermining trust in science, and that this is the last thing society needs right now.”
AI Makes Science Easier and More Accessible To Fake
Söderström highlighted that there are two main risks from this type of scientific flimflammery.
First is the increasing risk that undeclared and mischievous use of genAI in scholarly research produces believable—but still false—academic publications that can be tricky to detect.
Second, the sheer quantity of papers that large language models can produce suggests that the scholarly record risks being overwhelmed with bogus studies.
“One of our findings was that many of these papers have spread to several repositories online, and have appeared in social media. This is a common and mostly automated process, but it makes retractions or corrections of research extremely difficult. Especially because Google Scholar will keep on finding and displaying them,” he wrote.
AI Is Not To Blame —They Blame a Broken System
However, Söderström and his colleagues point out that AI itself isn’t the core problem it’s merely a tool that academicians have found to try and survive within the flawed “publish or perish” culture at most research universities.
The publishing of phony science papers is further compounded given Google’s disproportionate control over scholarly papers, search engines and basic access to online information.
He said the team is doing a larger, deeper dive on this specific topic since their initial query was so limited, but it turned up some many issues and left so many questions unanswered.
“While it is not clear that all papers were actually produced by individuals – they might also be produced by so-called paper mills producing results from fake studies resembling scholarly publications – there might be several potential reasons. The pressure for researchers to continuously publish scholarly output, which can be conducted more frequently through the use of LLM misconduct, could be one of the reasons,” Söderström expressed in the email.
The report doesn’t offer any simple solutions, but it does suggest a multi-pronged approach that needs to include technical, regulatory and educational components to protect the truth.
Let’s just hope we don’t have to wait much longer for it to get its boots on.
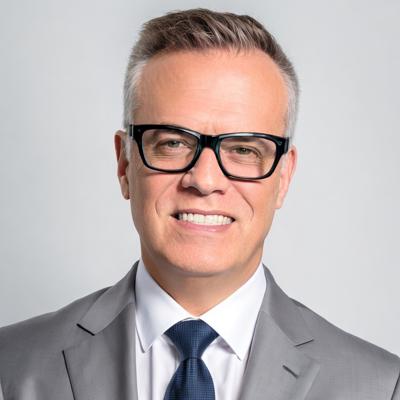
- Editorial Standards
- Reprints & Permissions
Join The Conversation
One Community. Many Voices. Create a free account to share your thoughts.
Forbes Community Guidelines
Our community is about connecting people through open and thoughtful conversations. We want our readers to share their views and exchange ideas and facts in a safe space.
In order to do so, please follow the posting rules in our site's Terms of Service. We've summarized some of those key rules below. Simply put, keep it civil.
Your post will be rejected if we notice that it seems to contain:
- False or intentionally out-of-context or misleading information
- Insults, profanity, incoherent, obscene or inflammatory language or threats of any kind
- Attacks on the identity of other commenters or the article's author
- Content that otherwise violates our site's terms.
User accounts will be blocked if we notice or believe that users are engaged in:
- Continuous attempts to re-post comments that have been previously moderated/rejected
- Racist, sexist, homophobic or other discriminatory comments
- Attempts or tactics that put the site security at risk
- Actions that otherwise violate our site's terms.
So, how can you be a power user?
- Stay on topic and share your insights
- Feel free to be clear and thoughtful to get your point across
- ‘Like’ or ‘Dislike’ to show your point of view.
- Protect your community.
- Use the report tool to alert us when someone breaks the rules.
Thanks for reading our community guidelines. Please read the full list of posting rules found in our site's Terms of Service.
Prenatal Development
- Reference work entry
- pp 1151–1152
- Cite this reference work entry
- Raymond S. Dean 3 &
- Renee Grizzle 3
1021 Accesses
Embryonic and fetal development
Prenatal development is defined as the process of growth and development within the womb from fertilization until birth. During this process, the zygote grows into an embryo and then a fetus.
Description
In the process of fertilization, a sperm cell from the male and the ovum (egg) of the female join in the female’s fallopian tube in conception. The prenatal development that occurs during the 38 weeks of pregnancy is typically separated into three periods: (1) the germinal period (the period of the zygote), (2) the embryonic period (the period of the embryo), and (3) the fetal period (the period of the fetus). A different way to describe the passage of time in pregnancy is to use 3-month time periods, immediately following conception, called trimesters [ 1 – 4 ].
The Germinal Period
The germinal period is the period of prenatal development that takes place in the first 2 weeks after conception. During this cell division, the fertilized...
This is a preview of subscription content, log in via an institution to check access.
Access this chapter
Subscribe and save.
- Get 10 units per month
- Download Article/Chapter or eBook
- 1 Unit = 1 Article or 1 Chapter
- Cancel anytime
- Available as PDF
- Read on any device
- Instant download
- Own it forever
- Available as EPUB and PDF
- Durable hardcover edition
- Dispatched in 3 to 5 business days
- Free shipping worldwide - see info
Tax calculation will be finalised at checkout
Purchases are for personal use only
Institutional subscriptions
Berk, L. E. (2006). Child development (7th ed.). Boston, MA: Pearson Education.
Google Scholar
Papalia, D. E., Gross, D., & Feldman, R. D. (2003). Child development: A topical approach . New York: McGraw-Hill.
Patterson, C. J. (2008). Child development . New York: McGraw-Hill.
Santrock, J. W. (2007). Child development (11th ed.). New York: McGraw-Hill.
Download references
Author information
Authors and affiliations.
Neuropsychology Laboratory - TC 904, Ball State University, Teachers College Room 502K, Muncie, IN, 47306, USA
Raymond S. Dean & Renee Grizzle
You can also search for this author in PubMed Google Scholar
Editor information
Editors and affiliations.
Neurology, Learning and Behavior Center, 230 South 500 East, Suite 100, Salt Lake City, Utah, 84102, USA
Sam Goldstein Ph.D.
Department of Psychology MS 2C6, George Mason University, Fairfax, VA, 22030, USA
Jack A. Naglieri Ph.D. ( Professor of Psychology ) ( Professor of Psychology )
Rights and permissions
Reprints and permissions
Copyright information
© 2011 Springer Science+Business Media, LLC
About this entry
Cite this entry.
Dean, R.S., Grizzle, R. (2011). Prenatal Development. In: Goldstein, S., Naglieri, J.A. (eds) Encyclopedia of Child Behavior and Development. Springer, Boston, MA. https://doi.org/10.1007/978-0-387-79061-9_2222
Download citation
DOI : https://doi.org/10.1007/978-0-387-79061-9_2222
Publisher Name : Springer, Boston, MA
Print ISBN : 978-0-387-77579-1
Online ISBN : 978-0-387-79061-9
eBook Packages : Behavioral Science Reference Module Humanities and Social Sciences Reference Module Business, Economics and Social Sciences
Share this entry
Anyone you share the following link with will be able to read this content:
Sorry, a shareable link is not currently available for this article.
Provided by the Springer Nature SharedIt content-sharing initiative
- Publish with us
Policies and ethics
- Find a journal
- Track your research

COMMENTS
The first embryo model generated from stem cells was published in 2014 and captured aspects of early human gastrulation 2. In a recent breakthrough, two papers in Nature, by Liu et al. 3 and Yu et ...
Early embryonic development is a complex process. The zygote undergoes several rounds of division to form a blastocyst, and during this process, the zygote undergoes the maternal-to-zygotic transition to gain control of embryonic development and makes two cell fate decisions to differentiate into an embryonic and two extra-embryonic lineages.
Zygote is an international journal dedicated to the rapid publication of original research in early embryology. It covers interdisciplinary studies in animals and humans, from gametogenesis through fertilization to gastrulation. The scope includes gametogenesis, sperm-oocyte interaction, gamete and embryo physiology, cell polarity, cell-cell ...
This article reviews the economical position that fertilization is the moment that personhood of the conceptus begins. Alternate positions proposing that personhood begins at other possible times after fertilization are presented and contrasted to the economical hypothesis. Keywords: Personhood, Zygote, Embryo, Fetus, Fertilization, Capacity.
ABSTRACT. Gene regulatory networks and tissue morphogenetic events drive the emergence of shape and function: the pillars of embryo development. Although model systems offer a window into the molecular biology of cell fate and tissue shape, mechanistic studies of our own development have so far been technically and ethically challenging.
There has been recent renewed interest in studying human early embryonic development. The advent of improved culture conditions to maintain blastocysts in vitro for an extended period and the emerging stem-cell-based models of the blastocyst and peri-implantation embryos have provided new information that is relevant to early human embryogenesis. However, the mechanism of lineage development ...
Zygote formation is an intricate and synchronized array of dynamic events, which is elicited by sperm and oocyte interaction (Niakan and Eggan 2013). Basically, it includes sperm penetration, subsequent calcium oscillations in oocytes followed by oocyte activation, sperm and oocyte fusion, development of male and female pronucleus, genome-wide ...
Abstract. Zygote, the first cell of the sporophytic generation, is a product of fertilization, the fusion of a female (egg cell) and male (sperm) gamete. After fertilization the zygote starts its characteristic type of development (embryogenesis) which, if continues undisturbed by external and inner factors, secures normal germination and post ...
The purpose of this chapter is to delineate embryo preimplantation development from the zygote stage to the blastocyst stage. This will begin with defining the critical developmental milestones, a discussion regarding novel methods of determining embryo viability (e.g. morphokinetics and metabolomics), and will conclude with a comparison ...
Zygote is an international journal dedicated to the rapid publication of original research in early embryology. It covers interdisciplinary studies in animals and humans, from gametogenesis through fertilization to gastrulation. The scope includes gametogenesis, sperm-oocyte interaction, gamete and embryo physiology, cell polarity, cell-cell ...
Recently, a research paper applying the approach to the human zygote has been published, and has led to the re-evaluation of the role of OCT4 (also known as POU5F1) in early human development (Fogarty et al., 2017).
Abstract. Purpose: To compare the effects of four culture media on the quality of human zygotes and embryos. Methods: Prospective study analyzing 2289 human embryos cultivated simultaneously in two different culture media: HTF, the default medium, with either Universal IVF, Global or IVF-30 as the secondary media.
Zygote is covered by the major abstracting and indexing services including Current Contents/Life Sciences, Chemical Abstracts and Index Medicus. Claims for missing issues should be made immediately on receipt of the subsequent issue.
The molecular basis of fertilization (Review) Fertilization is the fusion of the male and female gamete. The process involves the fusion of an oocyte with a sperm, creating a single diploid cell, the zygote, from which a new individual organism will develop. The elucidation of the molecular mechanisms of fertilization has fascinated researchers ...
The recent emergence of stem-cell-derived embryo models, a new field aiming to use stem cells to construct in vitro models to recapitulate snapshots of the development of the mammalian conceptus ...
The zygote (fertilized egg), through a series of well-defined developmental stages, forms an embryo (Fig. 11.1) the progenitor of the next generation. Several physical and chemical factors regulate the growth and development of the embryo. The surrounding tissues, especially the endosperm, also control the predetermined pattern of embryo ...
An international journal dedicated to the rapid publication of original research in early embryology, Zygote covers interdisciplinary studies on gametogenesis through fertilization to gastrulation in animals and humans. The scope has been expanded to include clinical papers, molecular and developmental genetics.
According to which zygote, embryo and fetus are human beings with all rights guaranteed to persons under their state constitutions (Miklavcic & Flaman, 2017).
These indicate that chromosome doubling of the haploid plant occurred at some point during development. Production and successful development of inter-subfamily wheat and rice double zygote (DZ) A zygote is a totipotent cell with a complete genome and cytoplasm equipped for proliferation.
Zygote. Zygote is an international journal dedicated to the rapid publication of original research in early embryology. It covers interdisciplinary studies in animals and humans, from gametogenesis through fertilization to gastrulation. The scope includes gametogenesis, sperm-oocyte interaction, gamete and embryo physiology, cell polarity ...
PDF | On Nov 27, 2019, F Muhjah and others published Fetal Development: Stages of Growth | Find, read and cite all the research you need on ResearchGate
In recent years, stem cell therapy has become a very promising and advanced scientific research topic. The development of treatment methods has evoked great expectations. This paper is a review focused on the discovery of different stem cells and the potential therapies based on these cells. The genesis of stem cells is followed by laboratory steps of controlled stem cell culturing and ...
New evidence supporting that fact comes from a group of Swedish researchers that just issued its findings regarding a growing number of fake scientific papers published to Google Scholar.The study ...
The prenatal development that occurs during the 38 weeks of pregnancy is typically separated into three periods: (1) the germinal period (the period of the zygote), (2) the embryonic period (the period of the embryo), and (3) the fetal period (the period of the fetus). A different way to describe the passage of time in pregnancy is to use 3 ...
Let's break this down step by step based on the example: 1. Example given: • Input: oyfjdnisdr rtqwainr acxz mynzbhhx • Output: Think step by step By examining the words: • The pattern involves selecting specific letters or transforming them. 2. Now, let's decode the new phrase: • Input: oyekaijzdf aaptcg suaokybhai ouow aqht mynznvaatzacdfoulxxz
Purpose: This paper is designed to critically review and analyze the body of research on a popular gang reduction strategy, implemented widely in the United States and a number of other countries, to: (1) assess whether researchers designed their evaluations to align with the theorized causal mechanisms that bring about reductions in violence; and (2) discuss how evidence on gang programs is ...