1.6 Mechanisms of Heat Transfer
Learning objectives.
By the end of this section, you will be able to:
- Explain some phenomena that involve conductive, convective, and radiative heat transfer
- Solve problems on the relationships between heat transfer, time, and rate of heat transfer
- Solve problems using the formulas for conduction and radiation
Just as interesting as the effects of heat transfer on a system are the methods by which it occurs. Whenever there is a temperature difference, heat transfer occurs. It may occur rapidly, as through a cooking pan, or slowly, as through the walls of a picnic ice chest. So many processes involve heat transfer that it is hard to imagine a situation where no heat transfer occurs. Yet every heat transfer takes place by only three methods:
- Conduction is heat transfer through stationary matter by physical contact. (The matter is stationary on a macroscopic scale—we know that thermal motion of the atoms and molecules occurs at any temperature above absolute zero.) Heat transferred from the burner of a stove through the bottom of a pan to food in the pan is transferred by conduction .
- Convection is the heat transfer by the macroscopic movement of a fluid. This type of transfer takes place in a forced-air furnace and in weather systems, for example.
- Heat transfer by radiation occurs when microwaves, infrared radiation, visible light, or another form of electromagnetic radiation is emitted or absorbed. An obvious example is the warming of Earth by the Sun. A less obvious example is thermal radiation from the human body.
In the illustration at the beginning of this chapter, the fire warms the snowshoers’ faces largely by radiation. Convection carries some heat to them, but most of the air flow from the fire is upward (creating the familiar shape of flames), carrying heat to the food being cooked and into the sky. The snowshoers wear clothes designed with low conductivity to prevent heat flow out of their bodies.
In this section, we examine these methods in some detail. Each method has unique and interesting characteristics, but all three have two things in common: there is a net transfer of heat solely because of a temperature difference, and the greater the temperature difference, the faster the heat transfer ( Figure 1.19 ).

Check Your Understanding 1.6
Name an example from daily life (different from the text) for each mechanism of heat transfer.
As you walk barefoot across the living room carpet in a cold house and then step onto the kitchen tile floor, your feet feel colder on the tile. This result is intriguing, since the carpet and tile floor are both at the same temperature. The different sensation is explained by the different rates of heat transfer: The heat loss is faster for skin in contact with the tiles than with the carpet, so the sensation of cold is more intense.
Some materials conduct thermal energy faster than others. Figure 1.20 shows a material that conducts heat slowly—it is a good thermal insulator, or poor heat conductor—used to reduce heat flow into and out of a house.
A molecular picture of heat conduction will help justify the equation that describes it. Figure 1.21 shows molecules in two bodies at different temperatures, T h T h and T c , T c , for “hot” and “cold.” The average kinetic energy of a molecule in the hot body is higher than in the colder body. If two molecules collide, energy transfers from the high-energy to the low-energy molecule. In a metal, the picture would also include free valence electrons colliding with each other and with atoms, likewise transferring energy. The cumulative effect of all collisions is a net flux of heat from the hotter body to the colder body. Thus, the rate of heat transfer increases with increasing temperature difference Δ T = T h − T c . Δ T = T h − T c . If the temperatures are the same, the net heat transfer rate is zero. Because the number of collisions increases with increasing area, heat conduction is proportional to the cross-sectional area—a second factor in the equation.
A third quantity that affects the conduction rate is the thickness of the material through which heat transfers. Figure 1.22 shows a slab of material with a higher temperature on the left than on the right. Heat transfers from the left to the right by a series of molecular collisions. The greater the distance between hot and cold, the more time the material takes to transfer the same amount of heat.
All four of these quantities appear in a simple equation deduced from and confirmed by experiments. The rate of conductive heat transfer through a slab of material, such as the one in Figure 1.22 , is given by
where P is the power or rate of heat transfer in watts or in kilocalories per second, A and d are its surface area and thickness, as shown in Figure 1.22 , T h − T c T h − T c is the temperature difference across the slab, and k is the thermal conductivity of the material. Table 1.5 gives representative values of thermal conductivity.
More generally, we can write
where x is the coordinate in the direction of heat flow. Since in Figure 1.22 , the power and area are constant, dT / dx is constant, and the temperature decreases linearly from T h T h to T c . T c .
Substance | Thermal Conductivity |
---|---|
Diamond | 2000 |
Silver | 420 |
Copper | 390 |
Gold | 318 |
Aluminum | 220 |
Steel iron | 80 |
Steel (stainless) | 14 |
Ice | 2.2 |
Glass (average) | 0.84 |
Concrete brick | 0.84 |
Water | 0.6 |
Fatty tissue (without blood) | 0.2 |
Asbestos | 0.16 |
Plasterboard | 0.16 |
Wood | 0.08–0.16 |
Snow (dry) | 0.10 |
Cork | 0.042 |
Glass wool | 0.042 |
Wool | 0.04 |
Polystyrene foam | 0.035 |
Down feathers | 0.025 |
Air | 0.023 |
Example 1.10
Calculating heat transfer through conduction.
k = 0.035 W/m · ° C k = 0.035 W/m · ° C for polystyrene foam; A = 0.950 m 2 ; A = 0.950 m 2 ; d = 2.50 cm = 0.0250 m; d = 2.50 cm = 0.0250 m; ; T c = 0 ° C; T c = 0 ° C; T h = 35.0 ° C T h = 35.0 ° C ; t = 1 day = 24 hours - 86,400 s. t = 1 day = 24 hours - 86,400 s.
Then we identify the unknowns. We need to solve for the mass of the ice, m . We also need to solve for the net heat transferred to melt the ice, Q . The rate of heat transfer by conduction is given by
The heat used to melt the ice is Q = m L f Q = m L f .We insert the known values:
Multiplying the rate of heat transfer by the time we obtain
We set this equal to the heat transferred to melt the ice, Q = m L f , Q = m L f , and solve for the mass m :
Significance
Table 1.5 shows that polystyrene foam is a very poor conductor and thus a good insulator. Other good insulators include fiberglass, wool, and goosedown feathers. Like polystyrene foam, these all contain many small pockets of air, taking advantage of air’s poor thermal conductivity.
In developing insulation , the smaller the conductivity k and the larger the thickness d , the better. Thus, the ratio d/k , called the R factor , is large for a good insulator. The rate of conductive heat transfer is inversely proportional to R . R factors are most commonly quoted for household insulation, refrigerators, and the like. Unfortunately, in the United States, R is still in non-metric units of ft 2 · °F · h/Btu ft 2 · °F · h/Btu , although the unit usually goes unstated [1 British thermal unit (Btu) is the amount of energy needed to change the temperature of 1.0 lb of water by 1.0 ° F 1.0 ° F , which is 1055.1 J]. A couple of representative values are an R factor of 11 for 3.5-inch-thick fiberglass batts (pieces) of insulation and an R factor of 19 for 6.5-inch-thick fiberglass batts ( Figure 1.23 ). In the US, walls are usually insulated with 3.5-inch batts, whereas ceilings are usually insulated with 6.5-inch batts. In cold climates, thicker batts may be used.
Note that in Table 1.5 , most of the best thermal conductors—silver, copper, gold, and aluminum—are also the best electrical conductors, because they contain many free electrons that can transport thermal energy. (Diamond, an electrical insulator, conducts heat by atomic vibrations.) Cooking utensils are typically made from good conductors, but the handles of those used on the stove are made from good insulators (bad conductors).
Example 1.11
Two conductors end to end.
We repeat the calculation with a second method, in which we use the thermal resistance R of the rod, since it simply adds when two rods are joined end to end. (We will use a similar method in the chapter on direct-current circuits.)
- Identify the knowns and convert them to SI units. The length of each rod is L A1 = L steel = 0.25 m, L A1 = L steel = 0.25 m, the cross-sectional area of each rod is A A1 = A steel = 7.85 × 10 −5 m 2 , A A1 = A steel = 7.85 × 10 −5 m 2 , the thermal conductivity of aluminum is k A1 = 220 W/m · ° C k A1 = 220 W/m · ° C , the thermal conductivity of steel is k steel = 80 W/m · ° C k steel = 80 W/m · ° C , the temperature at the hot end is T = 100 ° C T = 100 ° C , and the temperature at the cold end is T = 20 ° C T = 20 ° C .
- Calculate the heat-conduction rate through the steel rod and the heat-conduction rate through the aluminum rod in terms of the unknown temperature T at the joint: P steel = k steel A steel Δ T steel L steel = ( 80 W/m · ° C ) ( 7.85 × 10 −5 m 2 ) ( 100 ° C − T ) 0.25 m = ( 0.0251 W/ ° C ) ( 100 ° C − T ) ; P steel = k steel A steel Δ T steel L steel = ( 80 W/m · ° C ) ( 7.85 × 10 −5 m 2 ) ( 100 ° C − T ) 0.25 m = ( 0.0251 W/ ° C ) ( 100 ° C − T ) ; P A1 = k Al A A1 Δ T Al L A1 = ( 220 W/m · ° C ) ( 7.85 × 10 −5 m 2 ) ( T − 20 ° C ) 0.25 m = ( 0.0691 W/ ° C ) ( T − 20 ° C ) . P A1 = k Al A A1 Δ T Al L A1 = ( 220 W/m · ° C ) ( 7.85 × 10 −5 m 2 ) ( T − 20 ° C ) 0.25 m = ( 0.0691 W/ ° C ) ( T − 20 ° C ) .
- Set the two rates equal and solve for the unknown temperature: ( 0.0691 W/ ° C ) ( T − 20 ° C ) = ( 0.0251 W/ ° C ) ( 100 ° C − T ) T = 41.3 ° C . ( 0.0691 W/ ° C ) ( T − 20 ° C ) = ( 0.0251 W/ ° C ) ( 100 ° C − T ) T = 41.3 ° C .
- Calculate either rate: P steel = ( 0.0251 W/ ° C ) ( 100 ° C − 41.3 ° C ) = 1.47 W . P steel = ( 0.0251 W/ ° C ) ( 100 ° C − 41.3 ° C ) = 1.47 W .
- If desired, check your answer by calculating the other rate.
- Recall that R = L / k R = L / k . Now P = A Δ T / R , or Δ T = P R / A . P = A Δ T / R , or Δ T = P R / A .
- We know that Δ T steel + Δ T Al = 100 ° C − 20 ° C = 80 ° C Δ T steel + Δ T Al = 100 ° C − 20 ° C = 80 ° C . We also know that P steel = P Al , P steel = P Al , and we denote that rate of heat flow by P . Combine the equations: P R steel A + P R Al A = 80 ° C . P R steel A + P R Al A = 80 ° C . Thus, we can simply add R factors. Now, P = ( 80 ° C ) ( A ) R steel + R Al P = ( 80 ° C ) ( A ) R steel + R Al .
- Find the R s R s from the known quantities: R steel = 3.13 × 10 −3 m 2 · ° C/W R steel = 3.13 × 10 −3 m 2 · ° C/W and R Al = 1.14 × 10 −3 m 2 · ° C/W . R Al = 1.14 × 10 −3 m 2 · ° C/W .
- Substitute these values in to find P = 1.47 W P = 1.47 W as before.
- Determine Δ T Δ T for the aluminum rod (or for the steel rod) and use it to find T at the joint. Δ T Al = P R Al A = ( 1.47 W ) ( 1.14 × 10 −3 m 2 · ° C/W ) 7.85 × 10 −5 m 2 = 21.3 ° C, Δ T Al = P R Al A = ( 1.47 W ) ( 1.14 × 10 −3 m 2 · ° C/W ) 7.85 × 10 −5 m 2 = 21.3 ° C, so T = 20 ° C + 21.3 ° C = 41.3 ° C T = 20 ° C + 21.3 ° C = 41.3 ° C , as in Solution 1 1 .
- If desired, check by determining Δ T Δ T for the other rod.
Check Your Understanding 1.7
How does the rate of heat transfer by conduction change when all spatial dimensions are doubled?
Conduction is caused by the random motion of atoms and molecules. As such, it is an ineffective mechanism for heat transport over macroscopic distances and short times. For example, the temperature on Earth would be unbearably cold during the night and extremely hot during the day if heat transport into and through the atmosphere were only through conduction and radiation. Also, car engines would overheat unless there was a more efficient way to remove excess heat from the pistons. The next module discusses the important heat-transfer mechanism in such situations.
In convection , thermal energy is carried by the large-scale flow of matter. It can be divided into two types. In forced convection , the flow is driven by fans, pumps, and the like. A simple example is a fan that blows air past you in hot surroundings and cools you by replacing the air heated by your body with cooler air. A more complicated example is the cooling system of a typical car, in which a pump moves coolant through the radiator and engine to cool the engine and a fan blows air to cool the radiator.
In free or natural convection , the flow is driven by buoyant forces: hot fluid rises and cold fluid sinks because density decreases as temperature increases. The house in Figure 1.24 is kept warm by natural convection, as is the pot of water on the stove in Figure 1.25 . Ocean currents and large-scale atmospheric circulation, which result from the buoyancy of warm air and water, transfer hot air from the tropics toward the poles and cold air from the poles toward the tropics. (Earth’s rotation interacts with those flows, causing the observed eastward flow of air in the temperate zones.)
Interactive
Natural convection like that of Figure 1.24 and Figure 1.25 , but acting on rock in Earth’s mantle, drives plate tectonics that are the motions that have shaped Earth’s surface.
Convection is usually more complicated than conduction. Beyond noting that the convection rate is often approximately proportional to the temperature difference, we will not do any quantitative work comparable to the formula for conduction. However, we can describe convection qualitatively and relate convection rates to heat and time. Air is a poor conductor, so convection dominates heat transfer by air. Therefore, the amount of available space for airflow determines whether air transfers heat rapidly or slowly. There is little heat transfer in a space filled with air with a small amount of other material that prevents flow. The space between the inside and outside walls of a typical American house, for example, is about 9 cm (3.5 in.)—large enough for convection to work effectively. The addition of wall insulation prevents airflow, so heat loss (or gain) is decreased. On the other hand, the gap between the two panes of a double-paned window is about 1 cm, which largely prevents convection and takes advantage of air’s low conductivity reduce heat loss. Fur, cloth, and fiberglass also take advantage of the low conductivity of air by trapping it in spaces too small to support convection ( Figure 1.26 ).
Some interesting phenomena happen when convection is accompanied by a phase change. The combination allows us to cool off by sweating even if the temperature of the surrounding air exceeds body temperature. Heat from the skin is required for sweat to evaporate from the skin, but without air flow, the air becomes saturated and evaporation stops. Air flow caused by convection replaces the saturated air by dry air and evaporation continues.
Example 1.12
Calculating the flow of mass during convection.
We divide both sides of the equation by L v L v to find that the mass evaporated per unit time is
Another important example of the combination of phase change and convection occurs when water evaporates from the oceans. Heat is removed from the ocean when water evaporates. If the water vapor condenses in liquid droplets as clouds form, possibly far from the ocean, heat is released in the atmosphere. Thus, there is an overall transfer of heat from the ocean to the atmosphere. This process is the driving power behind thunderheads, those great cumulus clouds that rise as much as 20.0 km into the stratosphere ( Figure 1.27 ). Water vapor carried in by convection condenses, releasing tremendous amounts of energy. This energy causes the air to expand and rise to colder altitudes. More condensation occurs in these regions, which in turn drives the cloud even higher. This mechanism is an example of positive feedback, since the process reinforces and accelerates itself. It sometimes produces violent storms, with lightning and hail. The same mechanism drives hurricanes.
This time-lapse video shows convection currents in a thunderstorm, including “rolling” motion similar to that of boiling water.
Check Your Understanding 1.8
Explain why using a fan in the summer feels refreshing.
You can feel the heat transfer from the Sun. The space between Earth and the Sun is largely empty, so the Sun warms us without any possibility of heat transfer by convection or conduction. Similarly, you can sometimes tell that the oven is hot without touching its door or looking inside—it may just warm you as you walk by. In these examples, heat is transferred by radiation ( Figure 1.28 ). That is, the hot body emits electromagnetic waves that are absorbed by the skin. No medium is required for electromagnetic waves to propagate. Different names are used for electromagnetic waves of different wavelengths: radio waves, microwaves, infrared radiation, visible light, ultraviolet radiation, X-rays, and gamma rays.
The energy of electromagnetic radiation varies over a wide range, depending on the wavelength: A shorter wavelength (or higher frequency) corresponds to a higher energy. Because more heat is radiated at higher temperatures, higher temperatures produce more intensity at every wavelength but especially at shorter wavelengths. In visible light, wavelength determines color—red has the longest wavelength and violet the shortest—so a temperature change is accompanied by a color change. For example, an electric heating element on a stove glows from red to orange, while the higher-temperature steel in a blast furnace glows from yellow to white. Infrared radiation is the predominant form radiated by objects cooler than the electric element and the steel. The radiated energy as a function of wavelength depends on its intensity, which is represented in Figure 1.29 by the height of the distribution. ( Electromagnetic Waves explains more about the electromagnetic spectrum, and Photons and Matter Waves discusses why the decrease in wavelength corresponds to an increase in energy.)
The rate of heat transfer by radiation also depends on the object’s color. Black is the most effective, and white is the least effective. On a clear summer day, black asphalt in a parking lot is hotter than adjacent gray sidewalk, because black absorbs better than gray ( Figure 1.30 ). The reverse is also true—black radiates better than gray. Thus, on a clear summer night, the asphalt is colder than the gray sidewalk, because black radiates the energy more rapidly than gray. A perfectly black object would be an ideal radiator and an ideal absorber , as it would capture all the radiation that falls on it. In contrast, a perfectly white object or a perfect mirror would reflect all radiation, and a perfectly transparent object would transmit it all ( Figure 1.31 ). Such objects would not emit any radiation. Mathematically, the color is represented by the emissivity e . A “blackbody” radiator would have an e = 1 e = 1 , whereas a perfect reflector or transmitter would have e = 0 e = 0 . For real examples, tungsten light bulb filaments have an e of about 0.5, and carbon black (a material used in printer toner) has an emissivity of about 0.95.
To see that, consider a silver object and a black object that can exchange heat by radiation and are in thermal equilibrium. We know from experience that they will stay in equilibrium (the result of a principle that will be discussed at length in Second Law of Thermodynamics ). For the black object’s temperature to stay constant, it must emit as much radiation as it absorbs, so it must be as good at radiating as absorbing. Similar considerations show that the silver object must radiate as little as it absorbs. Thus, one property, emissivity, controls both radiation and absorption.
Finally, the radiated heat is proportional to the object’s surface area, since every part of the surface radiates. If you knock apart the coals of a fire, the radiation increases noticeably due to an increase in radiating surface area.
The rate of heat transfer by emitted radiation is described by the Stefan-Boltzmann law of radiation :
where σ = 5.67 × 10 −8 J/s · m 2 · K 4 σ = 5.67 × 10 −8 J/s · m 2 · K 4 is the Stefan-Boltzmann constant, a combination of fundamental constants of nature; A is the surface area of the object; and T is its temperature in kelvins.
The proportionality to the fourth power of the absolute temperature is a remarkably strong temperature dependence. It allows the detection of even small temperature variations. Images called thermographs can be used medically to detect regions of abnormally high temperature in the body, perhaps indicative of disease. Similar techniques can be used to detect heat leaks in homes ( Figure 1.32 ), optimize performance of blast furnaces, improve comfort levels in work environments, and even remotely map Earth’s temperature profile.
The Stefan-Boltzmann equation needs only slight refinement to deal with a simple case of an object’s absorption of radiation from its surroundings. Assuming that an object with a temperature T 1 T 1 is surrounded by an environment with uniform temperature T 2 T 2 , the net rate of heat transfer by radiation is
where e is the emissivity of the object alone. In other words, it does not matter whether the surroundings are white, gray, or black: The balance of radiation into and out of the object depends on how well it emits and absorbs radiation. When T 2 > T 1 , T 2 > T 1 , the quantity P net P net is positive (and when T 2 < T 1 , T 2 < T 1 , the quantity P net P net is negative), that is, the net heat transfer is from hot to cold.
Before doing an example, we have a complication to discuss: different emissivities at different wavelengths. If the fraction of incident radiation an object reflects is the same at all visible wavelengths, the object is gray; if the fraction depends on the wavelength, the object has some other color. For instance, a red or reddish object reflects red light more strongly than other visible wavelengths. Because it absorbs less red, it radiates less red when hot. Differential reflection and absorption of wavelengths outside the visible range have no effect on what we see, but they may have physically important effects. Skin is a very good absorber and emitter of infrared radiation, having an emissivity of 0.97 in the infrared spectrum. Thus, in spite of the obvious variations in skin color, we are all nearly black in the infrared. This high infrared emissivity is why we can so easily feel radiation on our skin. It is also the basis for the effectiveness of night-vision scopes used by law enforcement and the military to detect human beings.
Example 1.13
Calculating the net heat transfer of a person.
The average temperature of Earth is the subject of much current discussion. Earth is in radiative contact with both the Sun and dark space, so we cannot use the equation for an environment at a uniform temperature. Earth receives almost all its energy from radiation of the Sun and reflects some of it back into outer space. Conversely, dark space is very cold, about 3 K, so that Earth radiates energy into the dark sky. The rate of heat transfer from soil and grasses can be so rapid that frost may occur on clear summer evenings, even in warm latitudes.
The average temperature of Earth is determined by its energy balance. To a first approximation, it is the temperature at which Earth radiates heat to space as fast as it receives energy from the Sun.
An important parameter in calculating the temperature of Earth is its emissivity ( e ). On average, it is about 0.65, but calculation of this value is complicated by the great day-to-day variation in the highly reflective cloud coverage. Because clouds have lower emissivity than either oceans or land masses, they emit some of the radiation back to the surface, greatly reducing heat transfer into dark space, just as they greatly reduce heat transfer into the atmosphere during the day. There is negative feedback (in which a change produces an effect that opposes that change) between clouds and heat transfer; higher temperatures evaporate more water to form more clouds, which reflect more radiation back into space, reducing the temperature.
The often-mentioned greenhouse effect is directly related to the variation of Earth’s emissivity with wavelength ( Figure 1.33 ). The greenhouse effect is a natural phenomenon responsible for providing temperatures suitable for life on Earth and for making Venus unsuitable for human life. Most of the infrared radiation emitted from Earth is absorbed by carbon dioxide ( CO 2 CO 2 ) and water ( H 2 O H 2 O ) in the atmosphere and then re-radiated into outer space or back to Earth. Re-radiation back to Earth maintains its surface temperature about 40 °C 40 °C higher than it would be if there were no atmosphere. (The glass walls and roof of a greenhouse increase the temperature inside by blocking convective heat losses, not radiative losses.)
The greenhouse effect and its causes were first predicted by Eunice Newton Foote after she designed and conducted experiments on heating of different gases. After filling flasks with carbon dioxide, hydrogen, and regular air, then also modifying moisture, she placed them in the sun and carefully measured their heating and, especially, their heat retention. She discovered that the CO 2 flask gained the most temperature and held it the longest. After subsequent research, her paper "Circumstances affecting the Heat of the Sun’s Rays" included conclusions that an atmosphere consisting of more carbon dioxide would be hotter resulting from the gas trapping radiation.
The greenhouse effect is central to the discussion of global warming due to emission of carbon dioxide and methane (and other greenhouse gases) into Earth’s atmosphere from industry, transportation, and farming. Changes in global climate could lead to more intense storms, precipitation changes (affecting agriculture), reduction in rain forest biodiversity, and rising sea levels.
You can explore a simulation of the greenhouse effect that takes the point of view that the atmosphere scatters (redirects) infrared radiation rather than absorbing it and reradiating it. You may want to run the simulation first with no greenhouse gases in the atmosphere and then look at how adding greenhouse gases affects the infrared radiation from the Earth and the Earth’s temperature.
Problem-Solving Strategy
Effects of heat transfer.
- Examine the situation to determine what type of heat transfer is involved.
- Identify the type(s) of heat transfer—conduction, convection, or radiation.
- Identify exactly what needs to be determined in the problem (identify the unknowns). A written list is useful.
- Make a list of what is given or what can be inferred from the problem as stated (identify the knowns).
- Solve the appropriate equation for the quantity to be determined (the unknown).
- For conduction, use the equation P = k A Δ T d P = k A Δ T d . Table 1.5 lists thermal conductivities. For convection, determine the amount of matter moved and the equation Q = m c Δ T Q = m c Δ T , along with Q = m L f Q = m L f or Q = m L V Q = m L V if a substance changes phase. For radiation, the equation P net = σ e A ( T 2 4 − T 1 4 ) P net = σ e A ( T 2 4 − T 1 4 ) gives the net heat transfer rate.
- Substitute the knowns along with their units into the appropriate equation and obtain numerical solutions complete with units.
- Check the answer to see if it is reasonable. Does it make sense?
Check Your Understanding 1.9
How much greater is the rate of heat radiation when a body is at the temperature 40 ° C 40 ° C than when it is at the temperature 20 ° C 20 ° C ?
This book may not be used in the training of large language models or otherwise be ingested into large language models or generative AI offerings without OpenStax's permission.
Want to cite, share, or modify this book? This book uses the Creative Commons Attribution License and you must attribute OpenStax.
Access for free at https://openstax.org/books/university-physics-volume-2/pages/1-introduction
- Authors: Samuel J. Ling, William Moebs, Jeff Sanny
- Publisher/website: OpenStax
- Book title: University Physics Volume 2
- Publication date: Oct 6, 2016
- Location: Houston, Texas
- Book URL: https://openstax.org/books/university-physics-volume-2/pages/1-introduction
- Section URL: https://openstax.org/books/university-physics-volume-2/pages/1-6-mechanisms-of-heat-transfer
© Jul 23, 2024 OpenStax. Textbook content produced by OpenStax is licensed under a Creative Commons Attribution License . The OpenStax name, OpenStax logo, OpenStax book covers, OpenStax CNX name, and OpenStax CNX logo are not subject to the Creative Commons license and may not be reproduced without the prior and express written consent of Rice University.
- MyU : For Students, Faculty, and Staff
Science for All Outreach Program
Heat Flow Experiments
How does heat flow in solids?
Conduction: Popping balloons to explain conduction.
- Thermometer
- Water (hot, cold)
- Aluminum foil
- Stop watch (phone)
Convection: Use of food coloring agents in water currents to explain convection.
- Thermocouples and readers
- Tall beaker (1000 mL)
- Conical flask (25 mL)
- Food coloring agents
Radiation: Use of infrared cameras to study radiation
- Glass plate
- Plastic cover
- Glass slide
- Metal piece
Instructions:
Conduction:
- Inflate a balloon with air and ask the students what will happen if they bring the balloon near the candle. Also ask them to note down how long it takes for the balloon to pop.
- Now, ask the students to fill the balloon with ~10 mL of water (30 °C) and inflate with air. Ask them to hypothesize what will happen for this case?
- Discuss about good and bad conductors.
- Now repeat the experiment for different materials (cold water (30 °C), hot water (50 °C) aluminum foil, soil, corn syrup, glue)
Convection:
- Fill ¾th of the beaker with water at room temperature.
- Fill the conical flask with water and add ice. Mix well to get cold water and then add a food coloring agent
- Ask students to hypothesize what they will see if they place the flask in the beaker.
- Using tongs, carefully place the conical flask inside the beaker.
- Now ask the students to observe the convection current through color change.
- Discuss with the students about the density difference arising from temperature difference between water in the beaker and the conical flask.
- After some time, float an ice cube on water in the beaker and observe what happens.
- Repeat the experiment with water in conical flask at different temperatures (10 °C, 30 °C, 50 °C)
- Use a thermocouple to measure the temperature change with respect to beaker height.
- Repeat the experiment, except for this time, pour water from the conical flask into the beaker and observe the color change.
- Help students learn to use IR camera (focusing and taking pictures)
- Through IR camera, look at glass, Styrofoam and an object inside a plastic cover through IR camera. What do you observe? Talk about reflection, transmission and emission of thermal radiation.
- Cover a glass plate with the plastic cover in front of the IR camera. Position a candle behind the camera directly opposite to the glass plate. What do you see on the glass plate? This will help students understand that thermal radiation does not require medium to travel.
- Now ask the students to explore different objects with the camera
- Examples: Simultaneously eat glass and metal using a candle, Backside of a laptop during operation, Take selfies. Encourage them to take a cool picture, and we can project them at the end.
- Current Members
- Previous Members
- Funders and Partners
- Annual Reports
- School Teams
- AIMS 2015 - 2016
- AIMS 2016 - 2017
- About Andersen
- Andersen 2022 - 2023
- Andersen 2023 - 2024
- KIPP 2014 - 2015
- KIPP 2015 - 2016
- KIPP 2017 - 2018
- KIPP 2020 - 2021
- 2015 Field Trip
- 2016 Field Trip
- 2018 Field Trip
- 2022 Field Trip
- 2023 Field Trip
- 2024 Field Trip
- About Heritage Academy
- Heritage Academy 2021 - 2022
- Heritage Academy 2022 - 2023
- Heritage Academy 2023 - 2024
- About Marcy
- Marcy 2017 - 2018
- Marcy 2018 - 2019
- Marcy 2019 - 2020
- Marcy 2020 - 2021
- About Murray
- Murray 2018 - 2019
- Murray 2019 - 2020
- Murray 2020 - 2021
- Murray 2021 - 2022
- Murray 2022 - 2023
- Murray 2023 - 2024
- About Venture Academy
- Venture 2018 - 2019
- Venture 2019 - 2020
- Do It Yourself

- Science Notes Posts
- Contact Science Notes
- Todd Helmenstine Biography
- Anne Helmenstine Biography
- Free Printable Periodic Tables (PDF and PNG)
- Periodic Table Wallpapers
- Interactive Periodic Table
- Periodic Table Posters
- Science Experiments for Kids
- How to Grow Crystals
- Chemistry Projects
- Fire and Flames Projects
- Holiday Science
- Chemistry Problems With Answers
- Physics Problems
- Unit Conversion Example Problems
- Chemistry Worksheets
- Biology Worksheets
- Periodic Table Worksheets
- Physical Science Worksheets
- Science Lab Worksheets
- My Amazon Books
Heat Transfer – Conduction, Convection, Radiation

Heat transfer occurs when thermal energy moves from one place to another. Atoms and molecules inherently have kinetic and thermal energy, so all matter participates in heat transfer. There are three main types of heat transfer, plus other processes that move energy from high temperature to low temperature.
What Is Heat Transfer?
Heat transfer is the movement of heat due to a temperature difference between a system and its surroundings. The energy transfer is always from higher temperature to lower temperature, due to the second law of thermodynamics . The units of heat transfer are the joule (J), calorie (cal), and kilocalorie (kcal). The unit for the rate of heat transfer is the kilowatt (KW).
The Three Types of Heat Transfer With Examples
The three types of heat transfer differ according to the nature of the medium that transmits heat:
- Conduction requires contact.
- Convection requires fluid flow.
- Radiation does not require any medium.
- Conduction is heat transfer directly between neighboring atoms or molecules. Usually, it is heat transfer through a solid. For example, the metal handle of a pan on a stove becomes hot due to convection. Touching the hot pan conducts heat to your hand.
- Convection is heat transfer via the movement of a fluid, such as air or water. Heating water on a stove is a good example. The water at the top of the pot becomes hot because water near the heat source rises. Another example is the movement of air around a campfire. Hot air rises, transferring heat upward. Meanwhile, the partial vacuum left by this movement draws in cool outside air that feeds the fire with fresh oxygen.
- Radiation is the emission of electromagnetic radiation. While it occurs through a medium, it does not require one. For example, it’s warm outside on a sunny day because solar radiation crosses space and heats the atmosphere. The burner element of a stove also emits radiation. However, some heat from a burner comes from conduction between the hot element and a metal pan. Most real-life processes involve multiple forms of heat transfer.
Conduction requires that molecules touch each other, making it a slower process than convection or radiation. Atoms and molecules with a lot of energy have more kinetic energy and engage in more collisions with other matter. They are “hot.” When hot matter interacts with cold matter, some energy gets transferred during the collision. This drives conduction. Forms of matter that readily conduct heat are called thermal conductors .
Examples of Conduction
Conduction is a common process in everyday life. For example:
- Holding an ice cube immediately makes your hands feel cold. Meanwhile, the heat transferred from your skin to the ice melts it into liquid water.
- Walking barefoot on a hot road or sunny beach burns your feet because the solid material transmits heat into your foot.
- Iron clothes transfers heat from the iron to the fabric.
- The handle of a coffee cup filled with hot coffee becomes warm or even hot via conduction through the mug material.
Conduction Equation
One equation for conduction calculates heat transfer per unit of time from thermal conductivity, area, thickness of the material, and the temperature difference between two regions:
Q = [K ∙ A ∙ (T hot – T cold )] / d
- Q is heat transfer per unit time
- K is the coefficient of thermal conductivity of the substance
- A is the area of heat transfer
- T hot is the temperature of the hot region
- T cold is the temperature of the cold region
- d is the thickness of the body
Convection is the movement of fluid molecules from higher temperature to lower temperature regions. Changing the temperature of a fluid affects its density, producing convection currents. If the volume of a fluid increases, than its density decreases and it becomes buoyant.
Examples of Convection
Convection is a familiar process on Earth, primarily involving air or water. However, it applies to other fluids, such as refrigeration gases and magma. Examples of convection include:
- Boiling water undergoes convection as less dense hot molecules rise through higher density cooler molecules.
- Hot air rises and cooler air sinks and replaces it.
- Convection drives global circulation in the oceans between the equators and poles.
- A convection oven circulates hot air and cooks more evenly than one that only uses heating elements or a gas flame.
Convection Equation
The equation for the rate of convection relates area and the difference between the fluid temperature and surface temperature:
Q = h c ∙ A ∙ (T s – T f )
- Q is the heat transfer per unit time
- h c is the coefficient of convective heat transfer
- T s is the surface temperature
- T f is the fluid temperature
Radiation is the release of electromagnetic energy. Another name for thermal radiation is radiant heat. Unlike conduction or convection, radiation requires no medium for heat transfer. So, radiation occurs both within a medium (solid, liquid, gas) or through a vacuum.
Examples of Radiation
There are many examples of radiation:
- A microwave oven emits microwave radiation, which increases the thermal energy in food
- The Sun emits light (including ultraviolet radiation) and heat
- Uranium-238 emits alpha radiation as it decays into thorium-234
Radiation Equation
The Stephan-Boltzmann law describes relationship between the power and temperature of thermal radiation:
P = e ∙ σ ∙ A· (Tr – Tc) 4
- P is the net power of radiation
- A is the area of radiation
- Tr is the radiator temperature
- Tc is the surrounding temperature
- e is emissivity
- σ is Stefan’s constant (σ = 5.67 × 10 -8 Wm -2 K -4 )
More Heat Transfer – Chemical Bonds and Phase Transitions
While conduction, convection, and radiation are the three modes of heat transfer, other processes absorb and release heat. For example, atoms release energy when chemical bonds break and absorb energy in order to form bonds. Releasing energy is an exergonic process, while absorbing energy is an endergonic process. Sometimes the energy is light or sound, but most of the time it’s heat, making these processes exothermic and endothermic .
Phase transitions between the states of matter also involve the absorption or release of energy. A great example of this is evaporative cooling, where the phase transition from a liquid into a vapor absorbs thermal energy from the environment.
- Faghri, Amir; Zhang, Yuwen; Howell, John (2010). Advanced Heat and Mass Transfer . Columbia, MO: Global Digital Press. ISBN 978-0-9842760-0-4.
- Geankoplis, Christie John (2003). Transport Processes and Separation Principles (4th ed.). Prentice Hall. ISBN 0-13-101367-X.
- Peng, Z.; Doroodchi, E.; Moghtaderi, B. (2020). “Heat transfer modelling in Discrete Element Method (DEM)-based simulations of thermal processes: Theory and model development”. Progress in Energy and Combustion Science . 79: 100847. doi: 10.1016/j.pecs.2020.100847
- Welty, James R.; Wicks, Charles E.; Wilson, Robert Elliott (1976). Fundamentals of Momentum, Heat, and Mass Transfer (2nd ed.). New York: Wiley. ISBN 978-0-471-93354-0.
Related Posts
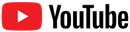
- TPC and eLearning
- What's NEW at TPC?
- Read Watch Interact
- Practice Review Test
- Teacher-Tools
- Request a Demo
- Get A Quote
- Subscription Selection
- Seat Calculator
- Ad Free Account
- Edit Profile Settings
- Metric Conversions Questions
- Metric System Questions
- Metric Estimation Questions
- Significant Digits Questions
- Proportional Reasoning
- Acceleration
- Distance-Displacement
- Dots and Graphs
- Graph That Motion
- Match That Graph
- Name That Motion
- Motion Diagrams
- Pos'n Time Graphs Numerical
- Pos'n Time Graphs Conceptual
- Up And Down - Questions
- Balanced vs. Unbalanced Forces
- Change of State
- Force and Motion
- Mass and Weight
- Match That Free-Body Diagram
- Net Force (and Acceleration) Ranking Tasks
- Newton's Second Law
- Normal Force Card Sort
- Recognizing Forces
- Air Resistance and Skydiving
- Solve It! with Newton's Second Law
- Which One Doesn't Belong?
- Component Addition Questions
- Head-to-Tail Vector Addition
- Projectile Mathematics
- Trajectory - Angle Launched Projectiles
- Trajectory - Horizontally Launched Projectiles
- Vector Addition
- Vector Direction
- Which One Doesn't Belong? Projectile Motion
- Forces in 2-Dimensions
- Being Impulsive About Momentum
- Explosions - Law Breakers
- Hit and Stick Collisions - Law Breakers
- Case Studies: Impulse and Force
- Impulse-Momentum Change Table
- Keeping Track of Momentum - Hit and Stick
- Keeping Track of Momentum - Hit and Bounce
- What's Up (and Down) with KE and PE?
- Energy Conservation Questions
- Energy Dissipation Questions
- Energy Ranking Tasks
- LOL Charts (a.k.a., Energy Bar Charts)
- Match That Bar Chart
- Words and Charts Questions
- Name That Energy
- Stepping Up with PE and KE Questions
- Case Studies - Circular Motion
- Circular Logic
- Forces and Free-Body Diagrams in Circular Motion
- Gravitational Field Strength
- Universal Gravitation
- Angular Position and Displacement
- Linear and Angular Velocity
- Angular Acceleration
- Rotational Inertia
- Balanced vs. Unbalanced Torques
- Getting a Handle on Torque
- Torque-ing About Rotation
- Properties of Matter
- Fluid Pressure
- Buoyant Force
- Sinking, Floating, and Hanging
- Pascal's Principle
- Flow Velocity
- Bernoulli's Principle
- Balloon Interactions
- Charge and Charging
- Charge Interactions
- Charging by Induction
- Conductors and Insulators
- Coulombs Law
- Electric Field
- Electric Field Intensity
- Polarization
- Case Studies: Electric Power
- Know Your Potential
- Light Bulb Anatomy
- I = ∆V/R Equations as a Guide to Thinking
- Parallel Circuits - ∆V = I•R Calculations
- Resistance Ranking Tasks
- Series Circuits - ∆V = I•R Calculations
- Series vs. Parallel Circuits
- Equivalent Resistance
- Period and Frequency of a Pendulum
- Pendulum Motion: Velocity and Force
- Energy of a Pendulum
- Period and Frequency of a Mass on a Spring
- Horizontal Springs: Velocity and Force
- Vertical Springs: Velocity and Force
- Energy of a Mass on a Spring
- Decibel Scale
- Frequency and Period
- Closed-End Air Columns
- Name That Harmonic: Strings
- Rocking the Boat
- Wave Basics
- Matching Pairs: Wave Characteristics
- Wave Interference
- Waves - Case Studies
- Color Addition and Subtraction
- Color Filters
- If This, Then That: Color Subtraction
- Light Intensity
- Color Pigments
- Converging Lenses
- Curved Mirror Images
- Law of Reflection
- Refraction and Lenses
- Total Internal Reflection
- Who Can See Who?
- Lab Equipment
- Lab Procedures
- Formulas and Atom Counting
- Atomic Models
- Bond Polarity
- Entropy Questions
- Cell Voltage Questions
- Heat of Formation Questions
- Reduction Potential Questions
- Oxidation States Questions
- Measuring the Quantity of Heat
- Hess's Law
- Oxidation-Reduction Questions
- Galvanic Cells Questions
- Thermal Stoichiometry
- Molecular Polarity
- Quantum Mechanics
- Balancing Chemical Equations
- Bronsted-Lowry Model of Acids and Bases
- Classification of Matter
- Collision Model of Reaction Rates
- Density Ranking Tasks
- Dissociation Reactions
- Complete Electron Configurations
- Elemental Measures
- Enthalpy Change Questions
- Equilibrium Concept
- Equilibrium Constant Expression
- Equilibrium Calculations - Questions
- Equilibrium ICE Table
- Intermolecular Forces Questions
- Ionic Bonding
- Lewis Electron Dot Structures
- Limiting Reactants
- Line Spectra Questions
- Mass Stoichiometry
- Measurement and Numbers
- Metals, Nonmetals, and Metalloids
- Metric Estimations
- Metric System
- Molarity Ranking Tasks
- Mole Conversions
- Name That Element
- Names to Formulas
- Names to Formulas 2
- Nuclear Decay
- Particles, Words, and Formulas
- Periodic Trends
- Precipitation Reactions and Net Ionic Equations
- Pressure Concepts
- Pressure-Temperature Gas Law
- Pressure-Volume Gas Law
- Chemical Reaction Types
- Significant Digits and Measurement
- States Of Matter Exercise
- Stoichiometry Law Breakers
- Stoichiometry - Math Relationships
- Subatomic Particles
- Spontaneity and Driving Forces
- Gibbs Free Energy
- Volume-Temperature Gas Law
- Acid-Base Properties
- Energy and Chemical Reactions
- Chemical and Physical Properties
- Valence Shell Electron Pair Repulsion Theory
- Writing Balanced Chemical Equations
- Mission CG1
- Mission CG10
- Mission CG2
- Mission CG3
- Mission CG4
- Mission CG5
- Mission CG6
- Mission CG7
- Mission CG8
- Mission CG9
- Mission EC1
- Mission EC10
- Mission EC11
- Mission EC12
- Mission EC2
- Mission EC3
- Mission EC4
- Mission EC5
- Mission EC6
- Mission EC7
- Mission EC8
- Mission EC9
- Mission RL1
- Mission RL2
- Mission RL3
- Mission RL4
- Mission RL5
- Mission RL6
- Mission KG7
- Mission RL8
- Mission KG9
- Mission RL10
- Mission RL11
- Mission RM1
- Mission RM2
- Mission RM3
- Mission RM4
- Mission RM5
- Mission RM6
- Mission RM8
- Mission RM10
- Mission LC1
- Mission RM11
- Mission LC2
- Mission LC3
- Mission LC4
- Mission LC5
- Mission LC6
- Mission LC8
- Mission SM1
- Mission SM2
- Mission SM3
- Mission SM4
- Mission SM5
- Mission SM6
- Mission SM8
- Mission SM10
- Mission KG10
- Mission SM11
- Mission KG2
- Mission KG3
- Mission KG4
- Mission KG5
- Mission KG6
- Mission KG8
- Mission KG11
- Mission F2D1
- Mission F2D2
- Mission F2D3
- Mission F2D4
- Mission F2D5
- Mission F2D6
- Mission KC1
- Mission KC2
- Mission KC3
- Mission KC4
- Mission KC5
- Mission KC6
- Mission KC7
- Mission KC8
- Mission AAA
- Mission SM9
- Mission LC7
- Mission LC9
- Mission NL1
- Mission NL2
- Mission NL3
- Mission NL4
- Mission NL5
- Mission NL6
- Mission NL7
- Mission NL8
- Mission NL9
- Mission NL10
- Mission NL11
- Mission NL12
- Mission MC1
- Mission MC10
- Mission MC2
- Mission MC3
- Mission MC4
- Mission MC5
- Mission MC6
- Mission MC7
- Mission MC8
- Mission MC9
- Mission RM7
- Mission RM9
- Mission RL7
- Mission RL9
- Mission SM7
- Mission SE1
- Mission SE10
- Mission SE11
- Mission SE12
- Mission SE2
- Mission SE3
- Mission SE4
- Mission SE5
- Mission SE6
- Mission SE7
- Mission SE8
- Mission SE9
- Mission VP1
- Mission VP10
- Mission VP2
- Mission VP3
- Mission VP4
- Mission VP5
- Mission VP6
- Mission VP7
- Mission VP8
- Mission VP9
- Mission WM1
- Mission WM2
- Mission WM3
- Mission WM4
- Mission WM5
- Mission WM6
- Mission WM7
- Mission WM8
- Mission WE1
- Mission WE10
- Mission WE2
- Mission WE3
- Mission WE4
- Mission WE5
- Mission WE6
- Mission WE7
- Mission WE8
- Mission WE9
- Vector Walk Interactive
- Name That Motion Interactive
- Kinematic Graphing 1 Concept Checker
- Kinematic Graphing 2 Concept Checker
- Graph That Motion Interactive
- Two Stage Rocket Interactive
- Rocket Sled Concept Checker
- Force Concept Checker
- Free-Body Diagrams Concept Checker
- Free-Body Diagrams The Sequel Concept Checker
- Skydiving Concept Checker
- Elevator Ride Concept Checker
- Vector Addition Concept Checker
- Vector Walk in Two Dimensions Interactive
- Name That Vector Interactive
- River Boat Simulator Concept Checker
- Projectile Simulator 2 Concept Checker
- Projectile Simulator 3 Concept Checker
- Hit the Target Interactive
- Turd the Target 1 Interactive
- Turd the Target 2 Interactive
- Balance It Interactive
- Go For The Gold Interactive
- Egg Drop Concept Checker
- Fish Catch Concept Checker
- Exploding Carts Concept Checker
- Collision Carts - Inelastic Collisions Concept Checker
- Its All Uphill Concept Checker
- Stopping Distance Concept Checker
- Chart That Motion Interactive
- Roller Coaster Model Concept Checker
- Uniform Circular Motion Concept Checker
- Horizontal Circle Simulation Concept Checker
- Vertical Circle Simulation Concept Checker
- Race Track Concept Checker
- Gravitational Fields Concept Checker
- Orbital Motion Concept Checker
- Angular Acceleration Concept Checker
- Balance Beam Concept Checker
- Torque Balancer Concept Checker
- Aluminum Can Polarization Concept Checker
- Charging Concept Checker
- Name That Charge Simulation
- Coulomb's Law Concept Checker
- Electric Field Lines Concept Checker
- Put the Charge in the Goal Concept Checker
- Circuit Builder Concept Checker (Series Circuits)
- Circuit Builder Concept Checker (Parallel Circuits)
- Circuit Builder Concept Checker (∆V-I-R)
- Circuit Builder Concept Checker (Voltage Drop)
- Equivalent Resistance Interactive
- Pendulum Motion Simulation Concept Checker
- Mass on a Spring Simulation Concept Checker
- Particle Wave Simulation Concept Checker
- Boundary Behavior Simulation Concept Checker
- Slinky Wave Simulator Concept Checker
- Simple Wave Simulator Concept Checker
- Wave Addition Simulation Concept Checker
- Standing Wave Maker Simulation Concept Checker
- Color Addition Concept Checker
- Painting With CMY Concept Checker
- Stage Lighting Concept Checker
- Filtering Away Concept Checker
- InterferencePatterns Concept Checker
- Young's Experiment Interactive
- Plane Mirror Images Interactive
- Who Can See Who Concept Checker
- Optics Bench (Mirrors) Concept Checker
- Name That Image (Mirrors) Interactive
- Refraction Concept Checker
- Total Internal Reflection Concept Checker
- Optics Bench (Lenses) Concept Checker
- Kinematics Preview
- Velocity Time Graphs Preview
- Moving Cart on an Inclined Plane Preview
- Stopping Distance Preview
- Cart, Bricks, and Bands Preview
- Fan Cart Study Preview
- Friction Preview
- Coffee Filter Lab Preview
- Friction, Speed, and Stopping Distance Preview
- Up and Down Preview
- Projectile Range Preview
- Ballistics Preview
- Juggling Preview
- Marshmallow Launcher Preview
- Air Bag Safety Preview
- Colliding Carts Preview
- Collisions Preview
- Engineering Safer Helmets Preview
- Push the Plow Preview
- Its All Uphill Preview
- Energy on an Incline Preview
- Modeling Roller Coasters Preview
- Hot Wheels Stopping Distance Preview
- Ball Bat Collision Preview
- Energy in Fields Preview
- Weightlessness Training Preview
- Roller Coaster Loops Preview
- Universal Gravitation Preview
- Keplers Laws Preview
- Kepler's Third Law Preview
- Charge Interactions Preview
- Sticky Tape Experiments Preview
- Wire Gauge Preview
- Voltage, Current, and Resistance Preview
- Light Bulb Resistance Preview
- Series and Parallel Circuits Preview
- Thermal Equilibrium Preview
- Linear Expansion Preview
- Heating Curves Preview
- Electricity and Magnetism - Part 1 Preview
- Electricity and Magnetism - Part 2 Preview
- Vibrating Mass on a Spring Preview
- Period of a Pendulum Preview
- Wave Speed Preview
- Slinky-Experiments Preview
- Standing Waves in a Rope Preview
- Sound as a Pressure Wave Preview
- DeciBel Scale Preview
- DeciBels, Phons, and Sones Preview
- Sound of Music Preview
- Shedding Light on Light Bulbs Preview
- Models of Light Preview
- Electromagnetic Radiation Preview
- Electromagnetic Spectrum Preview
- EM Wave Communication Preview
- Digitized Data Preview
- Light Intensity Preview
- Concave Mirrors Preview
- Object Image Relations Preview
- Snells Law Preview
- Reflection vs. Transmission Preview
- Magnification Lab Preview
- Reactivity Preview
- Ions and the Periodic Table Preview
- Periodic Trends Preview
- Chemical Reactions Preview
- Intermolecular Forces Preview
- Melting Points and Boiling Points Preview
- Bond Energy and Reactions Preview
- Reaction Rates Preview
- Ammonia Factory Preview
- Stoichiometry Preview
- Nuclear Chemistry Preview
- Gaining Teacher Access
- Task Tracker Directions
- Conceptual Physics Course
- On-Level Physics Course
- Honors Physics Course
- Chemistry Concept Builders
- All Chemistry Resources
- Users Voice
- Tasks and Classes
- Webinars and Trainings
- Subscription
- Subscription Locator
- 1-D Kinematics
- Newton's Laws
- Vectors - Motion and Forces in Two Dimensions
- Momentum and Its Conservation
- Work and Energy
- Circular Motion and Satellite Motion
- Thermal Physics
- Static Electricity
- Electric Circuits
- Vibrations and Waves
- Sound Waves and Music
- Light and Color
- Reflection and Mirrors
- Measurement and Calculations
- About the Physics Interactives
- Task Tracker
- Usage Policy
- Newtons Laws
- Vectors and Projectiles
- Forces in 2D
- Momentum and Collisions
- Circular and Satellite Motion
- Balance and Rotation
- Electromagnetism
- Waves and Sound
- Atomic Physics
- Forces in Two Dimensions
- Work, Energy, and Power
- Circular Motion and Gravitation
- Sound Waves
- 1-Dimensional Kinematics
- Circular, Satellite, and Rotational Motion
- Einstein's Theory of Special Relativity
- Waves, Sound and Light
- QuickTime Movies
- About the Concept Builders
- Pricing For Schools
- Directions for Version 2
- Measurement and Units
- Relationships and Graphs
- Rotation and Balance
- Vibrational Motion
- Reflection and Refraction
- Teacher Accounts
- Kinematic Concepts
- Kinematic Graphing
- Wave Motion
- Sound and Music
- About CalcPad
- 1D Kinematics
- Vectors and Forces in 2D
- Simple Harmonic Motion
- Rotational Kinematics
- Rotation and Torque
- Rotational Dynamics
- Electric Fields, Potential, and Capacitance
- Transient RC Circuits
- Light Waves
- Units and Measurement
- Stoichiometry
- Molarity and Solutions
- Thermal Chemistry
- Acids and Bases
- Kinetics and Equilibrium
- Solution Equilibria
- Oxidation-Reduction
- Nuclear Chemistry
- Newton's Laws of Motion
- Work and Energy Packet
- Static Electricity Review
- NGSS Alignments
- 1D-Kinematics
- Projectiles
- Circular Motion
- Magnetism and Electromagnetism
- Graphing Practice
- About the ACT
- ACT Preparation
- For Teachers
- Other Resources
- Solutions Guide
- Solutions Guide Digital Download
- Motion in One Dimension
- Work, Energy and Power
- Chemistry of Matter
- Names and Formulas
- Algebra Based On-Level Physics
- Honors Physics
- Conceptual Physics
- Other Tools
- Frequently Asked Questions
- Purchasing the Download
- Purchasing the Digital Download
- About the NGSS Corner
- NGSS Search
- Force and Motion DCIs - High School
- Energy DCIs - High School
- Wave Applications DCIs - High School
- Force and Motion PEs - High School
- Energy PEs - High School
- Wave Applications PEs - High School
- Crosscutting Concepts
- The Practices
- Physics Topics
- NGSS Corner: Activity List
- NGSS Corner: Infographics
- About the Toolkits
- Position-Velocity-Acceleration
- Position-Time Graphs
- Velocity-Time Graphs
- Newton's First Law
- Newton's Second Law
- Newton's Third Law
- Terminal Velocity
- Projectile Motion
- Forces in 2 Dimensions
- Impulse and Momentum Change
- Momentum Conservation
- Work-Energy Fundamentals
- Work-Energy Relationship
- Roller Coaster Physics
- Satellite Motion
- Electric Fields
- Circuit Concepts
- Series Circuits
- Parallel Circuits
- Describing-Waves
- Wave Behavior Toolkit
- Standing Wave Patterns
- Resonating Air Columns
- Wave Model of Light
- Plane Mirrors
- Curved Mirrors
- Teacher Guide
- Using Lab Notebooks
- Current Electricity
- Light Waves and Color
- Reflection and Ray Model of Light
- Refraction and Ray Model of Light
- Teacher Resources
- Subscriptions

- Newton's Laws
- Einstein's Theory of Special Relativity
- About Concept Checkers
- School Pricing
- Newton's Laws of Motion
- Newton's First Law
- Newton's Third Law
What is Heat?
- Introduction to Thermal Physics
- Temperature and Thermometers
- Thermometers as Speedometers
- Methods of Heat Transfer
- Rates of Heat Transfer
Earlier in this lesson , five dictionary style definitions of temperature were given. They were:
- The degree of hotness or coldness of a body or environment.
- A measure of the warmth or coldness of an object or substance with reference to some standard value.
- A measure of the average kinetic energy of the particles in a sample of matter, expressed in terms of units or degrees designated on a standard scale.
- A measure of the ability of a substance, or more generally of any physical system, to transfer heat energy to another physical system.
- Any of various standardized numerical measures of this ability, such as the Kelvin, Fahrenheit, and Celsius scale
As mentioned , the first two bullet points have rather obvious meanings. The third bullet point was the topic of the previous page in this lesson . The fifth bullet point was the definition that we started with as we discussed temperature and the operation of thermometers; it was the topic of the second page in this lesson . That leaves us with the fourth bullet point - defining temperature in terms of the ability of a substance to transfer heat to another substance. This part of Lesson 1 is devoted to understanding how the relative temperature of two objects affects the direction that heat is transferred between the two objects.
Consider a very hot mug of coffee on the countertop of your kitchen. For discussion purposes, we will say that the cup of coffee has a temperature of 80°C and that the surroundings (countertop, air in the kitchen, etc.) has a temperature of 26°C. What do you suppose will happen in this situation? I suspect that you know that the cup of coffee will gradually cool down over time. At 80°C, you wouldn't dare drink the coffee. Even the coffee mug will likely be too hot to touch. But over time, both the coffee mug and the coffee will cool down. Soon it will be at a drinkable temperature. And if you resist the temptation to drink the coffee, it will eventually reach room temperature. The coffee cools from 80°C to about 26°C. So what is happening over the course of time to cause the coffee to cool down? The answer to this question can be both macroscopic and particulate in nature.
Now let's consider a different scenario - that of a cold can of pop placed on the same kitchen counter. For discussion purposes, we will say that the pop and the can which contains it has a temperature of 5°C and that the surroundings (countertop, air in the kitchen, etc.) has a temperature of 26°C. What will happen to the cold can of pop over the course of time? Once more, I suspect that you know the answer. The cold pop and the container will both warm up to room temperature. But what is happening to cause these colder-than-room-temperature objects to increase their temperature? Is the cold escaping from the pop and its container? No! There is no such thing as the cold escaping or leaking . Rather, our explanation is very similar to the explanation used to explain why the coffee cools down. There is a heat transfer.
Another Definition of Temperature
Both of these scenarios could be summarized by two simple statements. An object decreases its temperature by releasing energy in the form of heat to its surroundings. And an object increases its temperature by gaining energy in the form of heat from its surroundings. Both the warming up and the cooling down of objects works in the same way - by heat transfer from the higher temperature object to the lower temperature object. So now we can meaningfully re-state the definition of temperature. Temperature is a measure of the ability of a substance, or more generally of any physical system, to transfer heat energy to another physical system. The higher the temperature of an object is, the greater the tendency of that object to transfer heat. The lower the temperature of an object is, the greater the tendency of that object to be on the receiving end of the heat transfer.
But perhaps you have been asking: what happens to the temperature of surroundings? Do the countertop and the air in the kitchen increase their temperature when the mug and the coffee cool down? And do the countertop and the air in the kitchen decrease its temperature when the can and its pop warm up? The answer is a resounding Yes! The proof? Just touch the countertop - it should feel cooler or warmer than before the coffee mug or pop can were placed on the countertop. But what about the air in the kitchen? Now that's a little more difficult to present a convincing proof of. The fact that the volume of air in the room is so large and that the energy quickly diffuses away from the surface of the mug means that the temperature change of the air in the kitchen will be abnormally small. In fact, it will be negligibly small . There would have to be a lot more heat transfer before there is a noticeable temperature change.
Thermal Equilibrium
In the discussion of the cooling of the coffee mug, the countertop and the air in the kitchen were referred to as the surroundings . It is common in physics discussions of this type to use a mental framework of a system and the surroundings . The coffee mug (and the coffee) would be regarded as the system and everything else in the universe would be regarded as the surroundings . To keep it simple, we often narrow the scope of the surroundings from the rest of the universe to simply those objects that are immediately surrounding the system. This approach of analyzing a situation in terms of system and surroundings is so useful that we will adopt the approach for the rest of this chapter and the next.
Now let's imagine a third situation. Suppose that a small metal cup of hot water is placed inside of a larger Styrofoam cup of cold water. Let's suppose that the temperature of the hot water is initially 70°C and that the temperature of the cold water in the outer cup is initially 5°C. And let's suppose that both cups are equipped with thermometers (or temperature probes) that measure the temperature of the water in each cup over the course of time. What do you suppose will happen? Before you read on, think about the question and commit to some form of answer. When the cold water is done warming and the hot water is done cooling, will their temperatures be the same or different? Will the cold water warm up to a lower temperature than the temperature that the hot water cools down to? Or as the warming and cooling occurs, will their temperatures cross each other ?
Fortunately, this is an experiment that can be done and in fact has been done on many occasions. The graph below is a typical representation of the results.
As you can see from the graph, the hot water cooled down to approximately 30°C and the cold water warmed up to approximately the same temperature. Heat is transferred from the high temperature object (inner can of hot water) to the low temperature object (outer can of cold water). If we designate the inner cup of hot water as the system , then we can say that there is a flow of heat from the system to the surroundings . As long as there is a temperature difference between the system and the surroundings, there is a heat flow between them. The heat flow is more rapid at first as depicted by the steeper slopes of the lines. Over time, the temperature difference between system and surroundings decreases and the rate of heat transfer decreases. This is denoted by the gentler slope of the two lines. (Detailed information about rates of heat transfer will be discussed later in this lesson .) Eventually, the system and the surroundings reach the same temperature and the heat transfer ceases. It is at this point, that the two objects are said to have reached thermal equilibrium .
The Zeroeth Law of Thermodynamics
In our chapter on electric circuits , we learned that a difference in electric potential between two locations causes a flow of charge along a conducting path between those locations. As long as an electric potential difference is maintained, a flow of charge will exist. Now in this chapter we learn a similar principle related to the flow of heat. A temperature difference between two locations will cause a flow of heat along a (thermally) conducting path between those two locations. As long as the temperature difference is maintained, a flow of heat will occur. This flow of heat continues until the two objects reach the same temperature. Once their temperatures become equal, they are said to be at thermal equilibrium and the flow of heat no longer takes place.
This principle is sometimes referred to as the zeroeth law of thermodynamics . This principle became formalized into a law after the first, second and third laws of thermodynamics had already been discovered . But because the law seemed more fundamental than the previously discovered three, it was titled the zeroeth law . All objects are governed by this law - this tendency towards thermal equilibrium. It represents a daily challenge for those who wish to control the temperature of their bodies, their food, their drinks and their homes. We use ice and insulation to try to keep our cold drinks cold and we use insulation and ongoing pulses of microwave energy to keep our hot drinks hot. We equip our vehicles, our homes and our office buildings equipped with air conditioners and fans in order to keep them cool during the warm summer months. And we equip these same vehicles and buildings with furnaces and heaters in order to keep them warm during the cold winter months. Whenever any of these systems are at a different temperature as the surroundings and not perfectly insulated from the surroundings (an ideal situation), heat will flow. This heat flow will continue until the system and surroundings have achieved equal temperatures. Because these systems have a considerably smaller volume than the surroundings, there will be a more noticeable and substantial change in temperature of these systems.
The Caloric Theory
Scientists have long pondered the nature of heat. Well into the mid-19th century, the most accepted notion of heat was one that associated it with a fluid known as caloric . Noted chemist Antoine Lavoisier reasoned that there were two forms of caloric - the kind that was latent or stored in combustible materials and the kind that was sensible and observable through a temperature change. For Lavoisier and his followers, the burning of fuel resulted in the release of this latent heat to the surroundings where it was observed to cause a temperature change of the surroundings. To Lavoisier and his followers, the heat was always present - either in latent form or in sensible form. If a hot kettle of water cooled down to room temperature, it was explained by the flow of caloric from the hot water to the surroundings.
The Fall of Caloric Theory
While there were always alternatives to the caloric theory, it was the most accepted view up until the mid 19th century. One of the first challenges to the caloric theory was from Anglo-American scientist Benjamin Thompson (a.k.a., Count Rumford). Thompson was one of the primary scientists appointed to the task of boring out the barrels of cannons for the British government. Thompson was amazed by the high temperatures reached by the cannons and by the shavings that were shed from the cannons during the boring process. In one experiment, he immersed the cannon in a tank of water during the boring process and observed that the heat generated by the boring process was capable of boiling the surrounding water within a few hours. Thompson demonstrated that this heat generation occurred in the absence of any chemical or physical change in the cannon's composition. He attributed the generation of heat to friction between the cannon and the boring tool and argued that it could not have been the result of the flow of fluid into the water. Thompson published a paper in 1798 that challenged the view that heat was a fluid that was conserved. He advocated a mechanical view of heat, suggesting that its origin was related to the motion of atoms and not the transfer of a fluid.
As we will learn in great detail in the next chapter, objects possess internal energy. In chemical reactions, a portion of this energy can be released to the surroundings in the form of heat. However, this internal energy is not a material substance or a fluid contained by the object. It is simply the potential energy stored in the bonds that hold particles within the object together. Heat or thermal energy is the form this energy possesses when it is being transferred between systems and surroundings . There is nothing material about heat. It is neither a substance nor a fluid that is conserved. Heat is a form of energy that can be transferred from one object to another or even created at the expense of the loss of other forms of energy.
To review, temperature is a measure of the ability of a substance, or more generally of any physical system, to transfer heat energy to another physical system. If two objects - or if a system and its surroundings - have a different temperature, then they have a different ability to transfer heat. Over time, there will be a flow of energy from the hotter object to the cooler object. This flow of energy is referred to as heat. The heat flow causes the hotter object to cool down and the colder object to warm up. The flow of heat will continue until they reach the same temperature. At this point, the two objects have established a thermal equilibrium with each other.
In the next part of this lesson , we will explore the mechanism of heat transfer. We will look at the various methods by which heat can be transferred from object to object or even from one location within an object to another. We will learn that the macroscopic can be explained in terms of the microscopic.
Check Your Understanding
1. For each of the following designations of a system and a surroundings, identify the direction of heat flow as being from the system to the surroundings or from the surroundings to the system.
Heat is the transfer of energy from a relatively hot location to a colder location. System Surroundings Dir'n of Heat Transfer a. Living Room (T=78°F) Outside Air (T=94°F) From surroundings to system b. Living Room (T=78°F) Attic (T=120°F) From surroundings to system c. Attic (T=120°F) Outside Air (T=94°F) From system to surroundings
2. A chemistry teacher claims that the heat content of a particular substance is 246 kJ/mol. Is the chemistry teacher claiming that the substance contains heat? Explain what it meant by this claim.
Answer: Hopefully not.
The chemistry teacher simply means that there are 246 kJ (kilojoules) of internal energy stored in the bonds between atoms in 1 mole of that substance. Chemistry types sometimes refer to this form of internal energy as enthalpy or chemical potential energy. Because it has the potential to be released in the form of heat during chemical reactions, chemistry teachers occasionally use the misnomer heat content to describe it. We all would be better served if we gave up the phrase heat content in favor of enthalpy or chemical potential energy or internal energy or ... .
3. Explain why high quality thermos bottles have a vacuum lining as a major component of their insulating ability.
Conduction and convection are heat transfer methods which depend upon the presence of materials to transfer heat. By lining a thermos bottle with a vacuum lining, energy cannot escape the contents of the bottle by two of the three forms of heat transfer.
- What Does Heat Do?
Cool Science Experiments Headquarters
Making Science Fun, Easy to Teach and Exciting to Learn!
Science Experiments
Conducting Heat Science Experiment
Which material conducts heat better, wood, plastic, or metal? In this experiment, we learn about conducting heat and how various materials conduct heat differently.
Note: Although the materials for this experiment are easy to find, one of the materials is boiling hot water. Depending on the age of your children the help of an adult is important. See our demonstration video and printable instructions below.
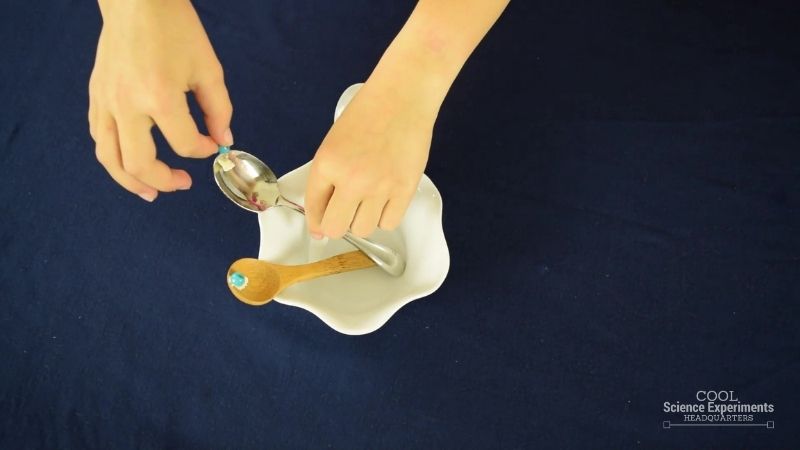
JUMP TO SECTION: Instructions | Video Tutorial | How it Works
Supplies Needed
- Small Glass Bowl
- Three Spoons (1 made out of wood, 1 made out of plastic and 1 made out of metal)
- Boiling Water
Conducting Heat Lab Kit – Only $5
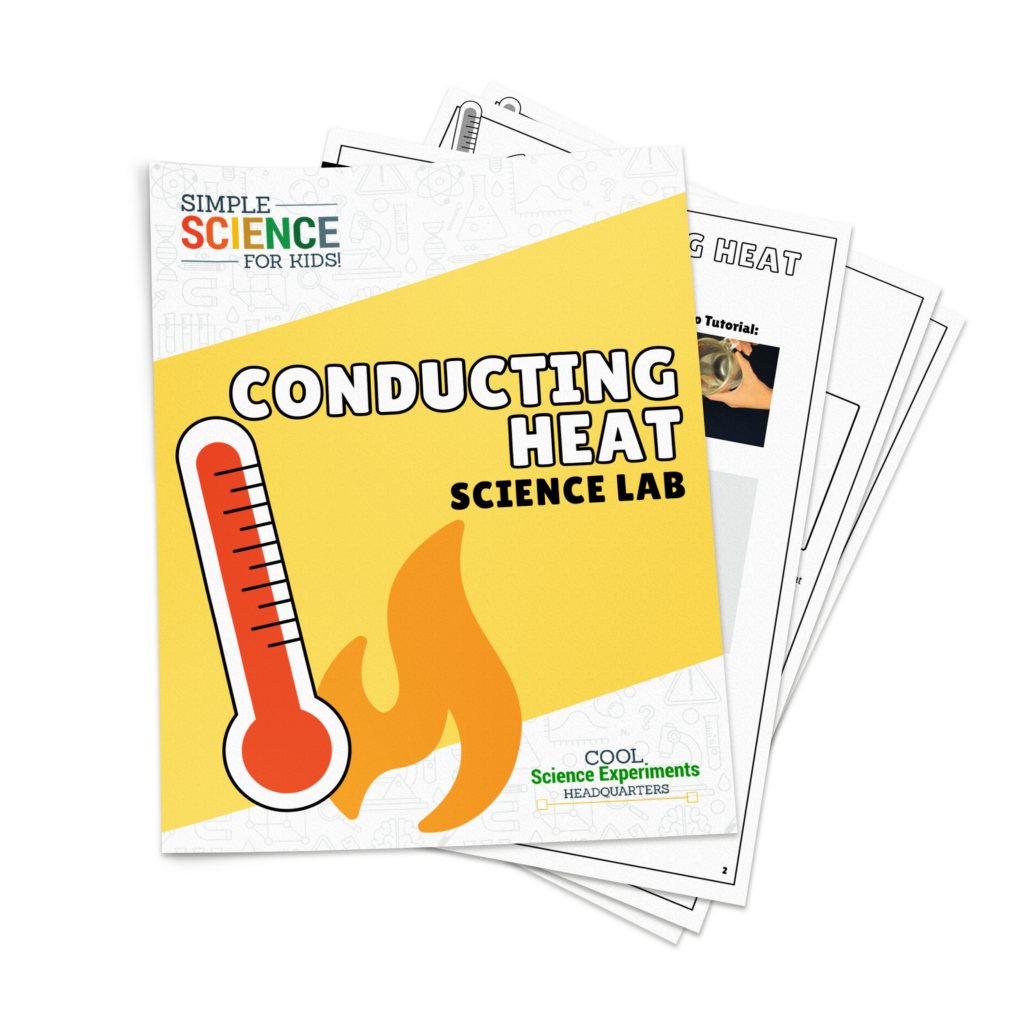
Use our easy Conducting Heat Science Lab Kit to grab your students’ attention without the stress of planning!
It’s everything you need to make science easy for teachers and fun for students — using inexpensive materials you probably already have in your storage closet!
Conducting Heat Science Experiment Instructions

Step 1 – Begin by positioning 3 spoons in a small glass bowl.

Step 2 – Place a small pat of butter at the top of each spoon.
Step 3 – Put a bead in each pat of butter.
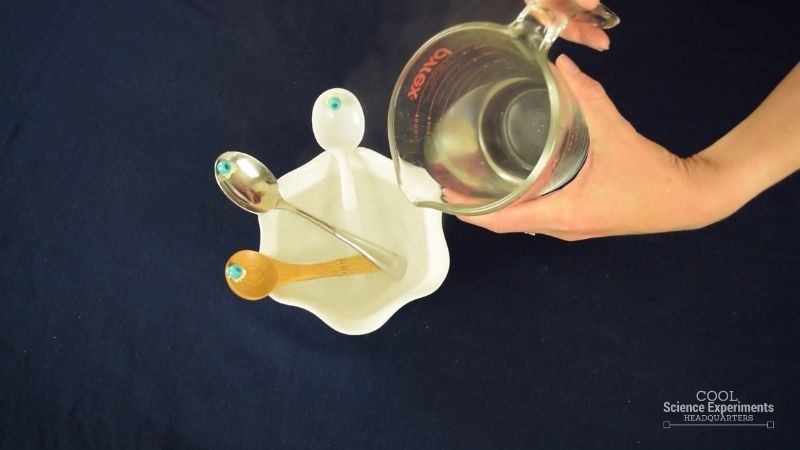
Step 4 – Carefully pour hot boiling water into the bowl until it is almost completely full. Be careful not to allow the spoons to fall into the bowl.
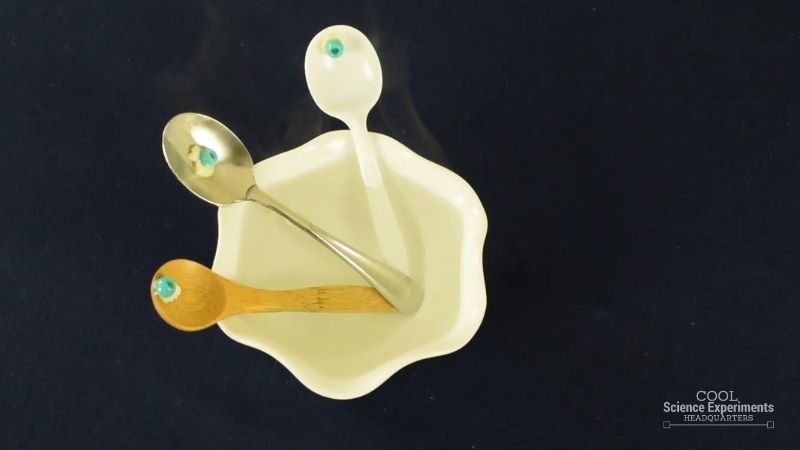
Step 5 – Watch carefully to see what happens to the beads. Write down your observations. Did all the beads behave the same? Do you know why? Find out the answer in the how does this experiment work section below.
Helpful Tip: You will likely need to watch the experiment for 5-10 minutes before anything happens.
Video Tutorial
How Does the Science Experiment Work
Heat can move in three ways: conduction, convection and radiation. In this experiment, the heat was transferred by means of conduction.
Conduction is the transfer of heat from one particle of matter to another without the movement of matter itself. As matter is heated, the particles that make up the matter begin to move faster.
In this experiment when we placed the spoons in the boiling water, the fast-moving water particles collide with the slow-moving spoon particles. As a result of the collision between the water particles and spoon particles, the particles of the spoon begin to move faster and the metal spoon becomes hotter. As the metal spoon gets hotter, the butter begins to melt and the bead slides down the spoon.
Why did the bead slide down the metal spoon faster than the wooden spoon or plastic spoon? Metal is a good conductor of heat, while wood and plastic are good insulators . A conductor transfers thermal energy (heat) well, while an insulator does not transfer thermal energy (heat) well.
I hope you enjoyed the experiment. Here are some printable instructions:
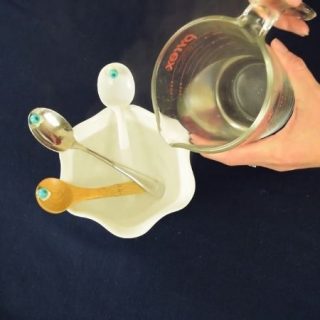
Instructions
- Begin my positioning 3 spoons in a small glass bowl.
- Place a small pat of butter at the top of each spoon
- Put a bead in each pat of butter
- Carefully pour hot boiling water into the bowl until it is almost completely full. Be careful not to allow the spoons to fall into the bowl.
- Watch carefully to see what happens to the beads. Note: You will likely need to watch the experiment for 5-10 minutes before anything happens.

Reader Interactions
March 5, 2019 at 6:35 am
Dear CoolScienceExperimentsHQ,
Thank you so much for sharing this, this really-really helped me and my group out on our science experiment on conduction and convection! Again i have to say thank you for this.
~a grateful 7th grader
May 5, 2023 at 7:53 pm
yes i agree with you this was very helpful and it looks fun to make.
May 10, 2023 at 3:59 am
same with me lol but for yr 8
February 2, 2024 at 11:42 am
I have not tried this experiment yet but, based on the comments so far I can be almost sure that it will work thanks for being super awesome scientists -A 5th grader that hopes to be grateful in the future
Leave a Reply Cancel reply
Your email address will not be published. Required fields are marked *
Save my name, email, and website in this browser for the next time I comment.
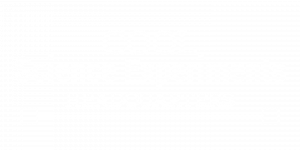
- Privacy Policy
- Disclosure Policy
Copyright © 2024 · Cool Science Experiments HQ
share this!
June 27, 2019
Experiment reverses the direction of heat flow
by José Tadeu Arantes, FAPESP
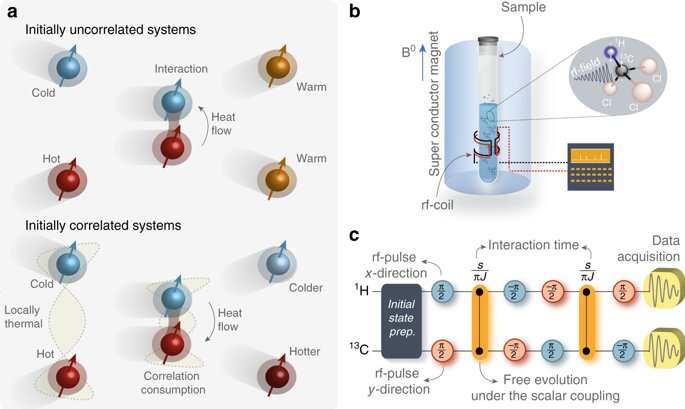
Heat flows from hot to cold objects. When a hot and a cold body are in thermal contact, they exchange heat energy until they reach thermal equilibrium, with the hot body cooling down and the cold body warming up. This is a natural phenomenon we experience all the time. It is explained by the second law of thermodynamics, which states that the total entropy of an isolated system always tends to increase over time until it reaches a maximum. Entropy is a quantitative measure of the disorder in a system. Isolated systems evolve spontaneously toward increasingly disordered states and lack of differentiation.
An experiment conducted by researchers at the Brazilian Center for Research in Physics (CBPF) and the Federal University of the ABC (UFABC), as well as collaborators at other institutions in Brazil and elsewhere, has shown that quantum correlations affect the way entropy is distributed among parts in thermal contact, reversing the direction of the so-called "thermodynamic arrow of time."
In other words, heat can flow spontaneously from a cold object to a hot object without the need to invest energy in the process, as is required by a domestic fridge. An article describing the experiment with theoretical considerations has just been published in Nature Communications .
The first author of the article, Kaonan Micadei, completed his Ph.D. under the supervision of Professor Roberto Serra and is now doing postdoctoral research in Germany. Serra, also one of the authors of the article, was supported by FAPESP via Brazil's National Institute of Science and Technology in Quantum Information. FAPESP also awarded two research grants linked to the project to another coauthor, Gabriel Teixeira Landi, a professor at the University of São Paulo's Physics Institute (IF-USP).
"Correlations can be said to represent information shared among different systems. In the macroscopic world described by classical physics, the addition of energy from outside can reverse the flow of heat in a system so that it flows from cold to hot. This is what happens in an ordinary refrigerator, for example," Serra told Agência FAPESP.
"It's possible to say that in our nanoscopic experiment, the quantum correlations produced an analogous effect to that of added energy. The direction of flow was reversed without violating the second law of thermodynamics. On the contrary, if we take into account elements of information theory in describing the transfer of heat, we find a generalized form of the second law and demonstrate the role of quantum correlations in the process."
The experiment was performed with a sample of chloroform molecules (a hydrogen atom, a carbon atom and three chlorine atoms) marked with a carbon-13 isotope. The sample was diluted in solution and studied using a nuclear magnetic resonance spectrometer, similar to the MRI scanners used in hospitals but with a much stronger magnetic field.
"We investigated temperature changes in the spins of the nuclei of the hydrogen and carbon atoms. The chlorine atoms had no material role in the experiment. We used radio frequency pulses to place the spin of each nucleus at a different temperature, one cooler, another warmer. The temperature differences were small, on the order of tens of billionths of 1 Kelvin, but we now have techniques that enable us to manipulate and measure quantum systems with extreme precision. In this case, we measured the radio frequency fluctuations produced by the atomic nuclei ," Serra said.
The researchers explored two situations: in one, the hydrogen and carbon nuclei began the process uncorrelated, and in the other, they were initially quantum-correlated.
"In the first case, with the nuclei uncorrelated, we observed heat flowing in the usual direction, from hot to cold, until both nuclei were at the same temperature. In the second, with the nuclei initially correlated, we observed heat flowing in the opposite direction, from cold to hot. The effect lasted a few thousandths of a second, until the initial correlation was consumed," Serra explained.
The most noteworthy aspect of this result is that it suggests a process of quantum refrigeration in which the addition of external energy (as is done in refrigerators and air conditioners to cool a specific environment) can be replaced by correlations, i.e., an exchange of information between objects.
Maxwell's demon
The idea that information can be used to reverse the direction of heat flow—in other words, to bring about a local decrease in entropy—arose in classical physics in the mid-nineteenth century, long before information theory was invented.
It was a thought experiment proposed in 1867 by James Clerk Maxwell (1831-1879), who, among other things, created the famous classical electromagnetism equations. In this thought experiment, which sparked a heated controversy at the time, the great Scottish physicist said that if there were a being capable of knowing the speed of each molecule of a gas and of manipulating all the molecules at the microscopic scale, this being could separate them into two recipients, placing faster-than-average molecules in one to create a hot compartment and slower-than-average molecules in the other to create a cold compartment. In this manner, a gas initially in thermal equilibrium owing to a mixture of faster and slower molecules would evolve to a differentiated state with less entropy.
Maxwell intended the thought experiment to prove that the second law of thermodynamics was merely statistical.
"The being he proposed, which was capable of intervening in the material world at the molecular or atomic scale, became known as "Maxwell's demon." It was a fiction invented by Maxwell to present his point of view. However, we're now actually able to operate at the atomic or even smaller scales, so that usual expectations are modified," Serra said.
The experiment conducted by Serra and collaborators and described in the article just published is a demonstration of this. It did not reproduce Maxwell's thought experiment , of course, but it produced an analogous result.
"When we talk about information, we're not referring to something intangible. Information requires a physical substrate, a memory. If you want to erase 1 bit of memory from a flash drive, you have to expend 10,000 times a minimum amount of energy consisting of the Boltzmann constant times the absolute temperature. This minimum of energy necessary to erase information is known as Landauer's principle. This explains why erasing information generates heat. Notebook batteries are consumed by heat more than anything else," Serra said.
What the researchers observed was that the information present in the quantum correlations can be used to perform work, in this case the transfer of heat from a colder to a hotter object, without consuming external energy.
"We can quantify the correlation of two systems by means of bits. Connections between quantum mechanics and information theory are creating what is known as quantum information science. From the practical standpoint, the effect we studied could one day be used to cool part of a quantum computer's processor," Serra said.
Journal information: Nature Communications
Provided by FAPESP
Explore further
Feedback to editors

Massive merger: Study reveals evidence for origin of supermassive black hole at galaxy's center
11 hours ago

Neolithic bones reveal isolated Yersinia pestis infections, not pandemics

New quantum error correction method uses 'many-hypercube codes' while exhibiting beautiful geometry

Solving the side effect problem of siRNA drugs for genetic disease treatment

Researchers advance new class of quantum critical metal that could advance electronic devices
12 hours ago

Protecting just 0.7% of world's land could help save a third of unique and endangered species
13 hours ago

Crystallized alternative DNA structure sheds light on insulin and diabetes

Researchers propose mechanistic framework to explain complex microbe-host symbioses

Low-cost nanomaterial technology can detect cancer genes with ultra-high sensitivity

Scientists uncover mechanism preserving centromere during cell division
Relevant physicsforums posts, how does output voltage of an electric guitar work.
3 hours ago
Looking for info on old, unlabeled Geissler tubes
Brownian motions and quantifying randomness in physical systems.
Sep 2, 2024
Container in an MRI room
Sep 1, 2024
Hysteresis of a Compressed Solid
Aug 30, 2024
Besides magnetic monopoles, what else doesn’t exist?
More from Other Physics Topics
Related Stories

Physicists implement a version of Maxwell's famous thought experiment for reducing entropy
Sep 5, 2018

Experiment shows that arrow of time is a relative concept, not an absolute one
Dec 1, 2017
Quantum correlations -- without entanglement
Aug 24, 2011

Maxwell's demon can use quantum information to generate work
Dec 18, 2013

Maxwell's demon demonstration turns information into energy
Nov 15, 2010

Quantum Maxwell's demon 'teleports' entropy out of a qubit
Dec 20, 2018

Recommended for you

Researchers make sound waves travel in one direction only, with implications for electromagnetic wave technology
14 hours ago

Why are black holes stable against their own gravity?
15 hours ago

First detection of cross-correlation between cosmic shear and X-ray background enhances baryonic matter understanding
Sep 5, 2024

Major leap for nuclear clock paves way for ultraprecise timekeeping
Sep 4, 2024

Bridging quantum mechanics and cosmology: The role of the generalized uncertainty principle

Supercomputer simulations provide new insights into calcium-48's controversial nuclear magnetic excitation
Sep 3, 2024
Let us know if there is a problem with our content
Use this form if you have come across a typo, inaccuracy or would like to send an edit request for the content on this page. For general inquiries, please use our contact form . For general feedback, use the public comments section below (please adhere to guidelines ).
Please select the most appropriate category to facilitate processing of your request
Thank you for taking time to provide your feedback to the editors.
Your feedback is important to us. However, we do not guarantee individual replies due to the high volume of messages.
E-mail the story
Your email address is used only to let the recipient know who sent the email. Neither your address nor the recipient's address will be used for any other purpose. The information you enter will appear in your e-mail message and is not retained by Phys.org in any form.
Newsletter sign up
Get weekly and/or daily updates delivered to your inbox. You can unsubscribe at any time and we'll never share your details to third parties.
More information Privacy policy
Donate and enjoy an ad-free experience
We keep our content available to everyone. Consider supporting Science X's mission by getting a premium account.
E-mail newsletter
Day 3, part 3: The heat flow experiment
Corrected transcript and commentary copyright © 2017-2023 by w. david woods and ben feist. all rights reserved..
Start of heat flow experiment | |
Film cycling in SIM bay cameras | |
Schmitt's report on food intake | |
Schmitt's weather report | |
Schmitt's exercise period | |
P52 platform realignment | |
Start of heat flow experiment during PTC | |
Schmitt's weather report | |
Stafford's conversation with Cernan | |
Photography of Earth | |
Last transmission on Day 3 |
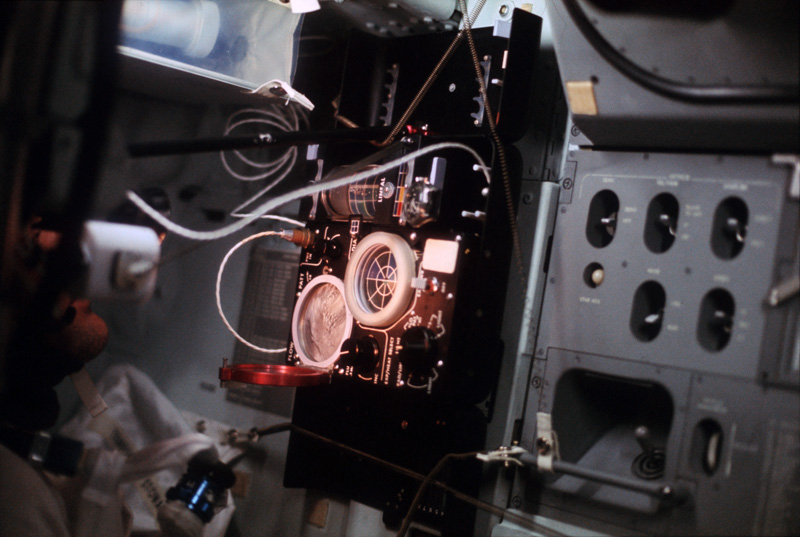
Physical Review Letters
- Collections
- Editorial Team
- Featured in Physics
- Editors' Suggestion
Experiments on Transformation Thermodynamics: Molding the Flow of Heat
Robert schittny, muamer kadic, sebastien guenneau, and martin wegener, phys. rev. lett. 110 , 195901 – published 10 may 2013, see focus story: invisibility cloak for heat.
- Citing Articles (475)
- Supplemental Material
- ACKNOWLEDGEMENTS
It was recently shown theoretically that the time-dependent heat conduction equation is form invariant under curvilinear coordinate transformations. Thus, in analogy to transformation optics, fictitious transformed space can be mapped onto (meta)materials with spatially inhomogeneous and anisotropic heat-conductivity tensors in the laboratory space. On this basis, we design, fabricate, and characterize a microstructured thermal cloak that molds the flow of heat around an object in a metal plate. This allows for transient protection of the object from heating while maintaining the same downstream heat flow as without object and cloak.
- Received 8 October 2012
DOI: https://doi.org/10.1103/PhysRevLett.110.195901
© 2013 American Physical Society
Invisibility Cloak for Heat
Published 10 may 2013.
Experimenters guide heat around a two-dimensional object without leaving a trace.
See more in Physics
Authors & Affiliations
- 1 Institute of Applied Physics, Karlsruhe Institute of Technology (KIT), 76128 Karlsruhe, Germany
- 2 Institut Fresnel, CNRS, Aix-Marseille Université, Campus Universitaire de Saint-Jérôme, 13013 Marseille, France
- 3 Institute of Nanotechnology, Karlsruhe Institute of Technology (KIT), 76128 Karlsruhe, Germany
Article Text (Subscription Required)
Supplemental material (subscription required), references (subscription required).
Vol. 110, Iss. 19 — 10 May 2013
Access Options
- Buy Article »
- Log in with individual APS Journal Account »
- Log in with a username/password provided by your institution »
- Get access through a U.S. public or high school library »
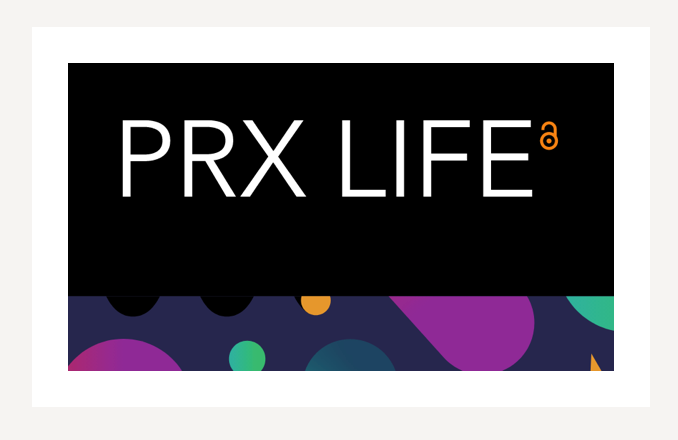
Authorization Required
Other options.
- Buy Article »
- Find an Institution with the Article »
Download & Share
Sign up to receive regular email alerts from Physical Review Letters
- Forgot your username/password?
- Create an account
Article Lookup
Paste a citation or doi, enter a citation.
Experimental Assessment of Multi-Phase Flow Distribution in an Evaporator Header Through Design of Experiments Techniques
41 Pages Posted: 5 Sep 2024
Claretta Tempesti
affiliation not provided to SSRN
Aude Lecardonnel
Delphine laboureur.
The uneven distribution of flow phases in evaporator channels can drop the heat exchanger efficiency up to 30%. Due to its dependence on the interaction of several coexisting variables - both geometry, operating conditions, and fluid properties - it is a complex phenomenon to analyse. Most studies focus on the effect of single parameters: this is an inefficient and expensive way of doing experiments, and the results lack in understanding how the combination of variables affects the flow distribution. This paper presents a methodology to optimally characterize and predict the distribution of flow phases in the channels of an evaporator header based on Design of Experiment (DoE) techniques. Despite the proven potential of DoE methods, they have never been applied in this field. Tests were conducted with an air-water mixture in the configuration horizontal header with vertical channels with downward flow, varying inlet pipe position, channels intrusion, presence of a splashing grid at the header inlet, and air and water flow rates. Results prove that, when working with complex processes, interaction effects between variables cannot be neglected as they significantly affect the response. The most affecting parameter was found to be the air flow rate, followed by the combination between inlet pipe position and presence of the splashing grid. With horizontal inlet, the optimal response was given by absence of intrusion, presence of the splashing grid, lowest water, and highest air flow rate. Instead, for the vertical case, the distribution was enhanced with the highest intrusion, absence of the grid, and highest water and air flow rates. Lastly a first attempt to model the process was performed. Even if a universal regression model has low accuracy (51%), restricting the area of analysis can result in valid predictive relations, with accuracies up to 91.4%.
Keywords: Design of Experiment, Applied statistical data analysis, experimental, Evaporator, Two-phase flow
Suggested Citation: Suggested Citation
Claretta Tempesti (Contact Author)
Affiliation not provided to ssrn ( email ).
No Address Available
Do you have a job opening that you would like to promote on SSRN?
Paper statistics, related ejournals, energy engineering ejournal.
Subscribe to this fee journal for more curated articles on this topic
Chemical Engineering eJournal
Environmental engineering ejournal.
Thank you for visiting nature.com. You are using a browser version with limited support for CSS. To obtain the best experience, we recommend you use a more up to date browser (or turn off compatibility mode in Internet Explorer). In the meantime, to ensure continued support, we are displaying the site without styles and JavaScript.
- View all journals
- Explore content
- About the journal
- Publish with us
- Sign up for alerts
- Open access
- Published: 27 August 2024
A numerical study of heat and mass transfer characteristic of three-dimensional thermally radiated bi-directional slip flow over a permeable stretching surface
- Hakeem Ullah 1 ,
- Syed Arshad Abas 1 ,
- Mehreen Fiza 1 ,
- Ilyas Khan 2 , 3 , 4 , 5 ,
- Ariana Abdul Rahimzai 6 &
- Ali Akgul 7 , 8
Scientific Reports volume 14 , Article number: 19842 ( 2024 ) Cite this article
262 Accesses
Metrics details
- Engineering
- Mathematics and computing
Within fluid mechanics, the flow of hybrid nanofluids over a stretching surface has been extensively researched due to their influence on the flow and heat transfer properties. Expanding on this concept by introducing porous media, the current study explore the flow and heat and mass transport characteristics of hybrid nanofluid. This investigation includes the effect of magnetohydrodynamic (MHD) with chemical reaction, thermal radiation, and slip effects. The nanoparticles, copper, and alumina are combined with water for the formation of a hybrid nanofluid. Using the self-similar method for the reduction of Partial differential equations (PDEs) to the system of Ordinary differential equations (ODEs). These nonlinear equation systems are solved numerically using the bvp4c (boundary value solver) technique. The effect of the different physical non-dimensional flow parameters on different flow profiles such as velocity, temperature, concentration, skin friction, Nusselt and mass transfer rate are depicted through graphs and tables. The velocity profiles diminish with the effect of magnetic and slip parameters. The temperature and concentration slip parameters reduce the temperature and concentration profile respectively. The higher values of magnetic factor lessened the skin friction coefficient for both slip and no-slip conditions. An elevation in the thermal slip parameter reduced the boundary layer thickness and the heat transfer from the surface to the fluid. The Nusselt number amplified with the climbing values of the radiation parameter. The mass transfer rate depressed with the solutal slip parameter. Comparison is made with the published work in the literature and there is excellent agreement between them.
Similar content being viewed by others
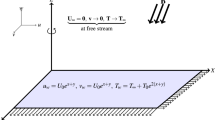
Exploration of the effects of Coriolis force and thermal radiation on water-based hybrid nanofluid flow over an exponentially stretching plate
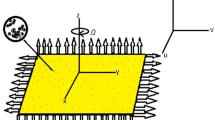
Transportation of thermal and velocity slip factors on three-dimensional dual phase nanomaterials liquid flow towards an exponentially stretchable surface
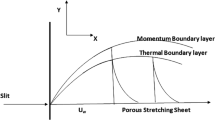
Two-dimensional nanofluid flow impinging on a porous stretching sheet with nonlinear thermal radiation and slip effect at the boundary enclosing energy perspective
Introduction.
The exploration of nanofluid has expanded significantly among researchers due to its wide-ranging significance in various fields such as biomedicine, cooling for electronic devices, transportation, heat exchangers, and many more. Diverse nanoparticles such as grapheme, silver, copper, alumina, silica, and carbon nanotube must be incorporated into the base fluid to enhance the thermal conductivity of common fluids like ethylene glycol water, kerosene, and engine oils. The utilization of nanotechnology in industry had become prevalent due to the distinctive physical and chemical properties exhibited by materials at the nanometer scale. Fluids containing nano-scale particles are called nanofluids, a term initially coined by Choi 1 . In their work, Choi et al. 2 demonstrated that incorporating a small quantity of less than 1% by volume of nanoparticles into conventional heat transfer liquids resulted in a notable enhancement of the fluid's thermal conductivity reaching approximately twice its original value. Vedavathi et al. 3 examined the Darcy nanofluid over an enlarging surface with thermal radiation and chemical reaction numerically. They concluded that with the heightened values of magnetic and Darcy number, the velocity profile declined while the temperature graphs boosted. The demand to enhance the thermal performance of the fluids in science and technology a new class of fluid called hybrid nanofluids was utilized. The formation of hybrid nanofluid involves more than one nanoparticle in a base fluid. Abas et al. 4 studied the Cu and alumina blood-base hybrid nanofluid with thermophoresis and Arrhenius activation energy. They concluded that the concentration graph raised with activation and thermophoresis parameters. Additionally, the rate of heat transport is more pronounced than nanofluid. Propylene glycol hybrid nanofluid two-dimensional Williamson fluid flow over a convectively heated porous surface examined by Ali et al. 5 . They revealed that the heat transfer rate of hybrid nanofluid is higher, compared to other regular fluids. Moreover, the temperature and entropy profile amplified with radiation factor. Saeed et al. 6 examined Darcy Forchheimer hybrid nanofluid flow having carbon nanotubes and iron oxide past an exponentially extending curved surface. They found that the rate of heat transfer increased by 3.1461% more than other fluids. Guedri et al. 7 studied the two-dimensional ternary hybrid nanofluid over an extended surface with entropy generation. The higher values of both the electric and magnetic parameters promoted the Bejan number while hindering the generation of entropy. Dawar et al. 8 studied the magnetized and non-magnetize non-Newtonian flow over a stretchy cylinder. They marked that the intensified values of \(\beta \to \infty\) the velocity graph escalated, on the other hand \(\beta \to 1.0\) velocity lines curtailed. Zangooee et al. 9 used the fifth-order RKF method for the solution of hybrid nanofluid across a two directional enlarging surface with non-uniform magnetic field. They concluded that the temperature had direct linked with the enhancement of the shape factor. Rehman et al. 10 examined the application of hybrid nanofluids in industries to enhance their thermal performance. They noted that both the magnetic and Eckert number boost the temperature profile. The investigation of the fluid flow over an extended surface was pertinent to several key applications in engineering, particularly within the domains of metallurgy and chemical engineering processes. These uses frequently revolved around the cooling of continuous strips or filaments by drawing them through a quiescent fluid. The research conducted by Crane 11 probed into the examination of the steady two-dimensional boundary layer Newtonian fluid flow over a surface undergoing stretching. This exploration contributed valuable insights into the understanding of relevant phenomena in these industrial processes. After Crane the attention of many scientists drawn toward the problem of stretching surface. Homotopic modeling of mixed-mode high-velocity bio-convective flow in a water-based hybrid nanofluid across a thermal convective exponentially expanding structure examined by Shamshuddin et al. 12 . Das 13 studied the slip effect on non-linear stretching surface. The finding revealed that the boundary layer thickness and stream-wise velocity enrich with the enhanced value of the stretching factor. Numerically deliberated the MHD steady flow over a stretchy surface by 14 . Their result was in excellent agreement with the previous work. Also, the heat transfer rate raised with Hartman number and stretching ratio factors. Waqas et al. 15 studied the effect of thermal radiation in the presence of a heat source on hybrid nanofluid over an extended surface. The growing values of the thermal radiation declared the higher profile for temperature while diminishing for Deborah number. By imposing innovative bilateral reactions, Shamshuddin et al. 16 builted a model of a two-phase nanofluid flowing through a bidirectional stretching sheet. The findings showed that a higher stretching value increased the nanofluid's velocity, whereas a lower Casson parameter decreased the x and y velocities. Bouslimi et al. 17 numerically studied the thermally radiated Williamson nanofluid over a permeable stretching surface in the existence of heat generation and absorption using the fourth-order Rung-Kutta method. They found that the Nusselt number decreased with the augmented heat generation and absorption coefficient values. Furthermore, the skin friction declined with magnetic and Darcy numbers. The work was carried out by Lone et al. 18 to examined the Casson hybrid nanofluid flow induced by external forces as it passed an exponentially extending surface. Extensive analysis of the data showed that as magnetic, mixed convection, buoyancy ratio, Casson, and porosity parameters escalated, fluid motion deteriorated. The literature 19 , 20 , 21 investigated the MHD flow over a permeable and non-permeable stretching surface. Sajid et al. 22 investigated numerically micro polar flow over a curved stretching surface by Runge- Kutta method. They found that the drag force is more for flat surfaces as compared to curved surfaces. Additionally, a curved sheet has a thicker boundary layer than a flat sheet.
Magnetohydrodynamics is a field of investigation that examined the dynamics of electrically conductive materials. The term comprises magneto for magnetic effects hydro for water and dynamic for movement. In this phenomena forces arise from magnetic fields induced by currents. Researchers have been significantly drawn his attention to this field in recent decades. MHD played a crucial role in geophysics engineering and astrophysics. Notably, it contributes to the cooling of nuclear reactors using liquid metals and the confinement of plasma. Aerospace vehicles also benefit from the application of MHD. Kumar et al. 23 evaluated natural convection within MHD flow where an accelerated plate was taken into account along with the effects of Hall current and Soret-Dufour. The mass transfer rate grows with the Schmidt number. To explored the impact of activation energy Reddy et al. 24 examined the radiative MHD nanofluid over a porous wedge. They disclosed that the boundary layer thickness expands with the growing of activation energy. Furthermore, the Nusselt number declined with the Eckert and thermophoresis parameters. Ali et al. 25 scrutinized the MHD flow with variable thickness of the extended sheet containing TiO 2 and copper nanoparticles. They used the Cattaneo-Christov heat flux model to enhance the thermal performance. They concluded that temperature amplified with nanoparticle volume percentage. The thermally radiative mixed convected hybrid nanofluid flow studied by Vishalaskshi et al. 26 past over an extending and shrinking surface with a dual effect of inclined MHD. The velocity was the growing function of Rayleigh number. Naveed et al. 27 used curvilinear coordinates to examine the MHD flow over an extended curved surface. They noted that the unsteadiness parameter suppresses both pressure and momentum boundary layer thickness.
The presence of slip boundary conditions is defined by a correlation between the tangential velocity at the surface and the shear stress applied to the wall. Navier introduced the term slip boundary condition after establishing a connection between slip rate and shear stress. Maxwell provided a straightforward explanation for velocity slip, attributing it to a change in velocity parallel to both the creep term and the surface. Thermal slip involves temperature variations along a surface with a specific temperature and concentration slip refers to the alteration in the current concentration due to the introduction of a new solute concentration. Andersson 28 conducted earlier studies that considered the slip boundary Newtonian flow over an extended surface. He presented a comprehensive closed-form solution for the Navier–Stokes equations addressing MHD flow over a stretching sheet. Subsequently the Andersson contribution, Wang 29 further advanced the field by unveiling a closed-form solution similarity solution for complete Navier–Stokes equations specifically for the flow over a stretching sheet with partial slip. Hayat et al. 30 extended the work of previous researchers by introducing thermal slip conditions. They explored the dynamic of unsteady MHD flow and heat transfer over a sponge-extended surface incorporating slip conditions. Sharma et al. 31 numerically examined the slip effect on micro polar flow over an extended porous surface. The slip and porous factors cut down the velocity profile. Ali et al. 32 explored microorganism Darcy Forchheimer’s nanofluid flow with slip condition over a spinning disk. They used both numerical and analytical method for the computating of the flow model. Raza et al. 33 studied the heat and mass transfer characteristic of three-dimensional MHD flow considering the effects of the slip across a rotating channel. The provided references 34 , 35 , 36 , 37 contain the investigation and findings of the slips’ effects on different flow problems.
This study aims to examine MHD hybrid nanofluid over a bi-directional permeable stretching sheet considering slip flow and heat transport of the hybrid nanofluid ( \(Cu + Al_{2} O_{3} /H_{2} O\) ) having nanoparticles \(Cu\) and \(Al_{2} O_{3}\) are suspended in the base fluid water. It is well-known that these nanoparticles have many engineering applications, including cosmetics, the automobile industry, the home industry, cancer therapy, food packaging, medicines, textiles, paper, plastics, paints, ceramics, food colourants, soaps, and many more. The core of the ongoing proposed model is to analyze the effects of different slips factors on the flow and heat transmission behavior of water base hybrid nanofluid. The proposed model of the \(Cu + Al_{2} O_{3} /H_{2} O\) nanofluid is still not being study by the researcher with such configured effects based on the cited literature. The governing PDEs are transformed into ODEs and subsequently solved numerically. To validate the numerical approach the results are compared with those of Hayat et al. 38 for different \(\beta\) values. Additionally, the study explores the influence of a strong magnetic field, thermophoresis, Brownian motion, thermal radiation, and chemical reaction. Furthermore, the effects of different non-dimensional parameters on flow profile and rate of heat and mass transfer are examined.
Mathematical model
Consider a steady MHD hybrid nanofluid three-dimensional flow over a stretching surface. Assume that the sheet is porous and stretched in two directions i.e. \(x_{1} -\) and \(x_{2} -\) as shown in (Fig. 1 ). The coordinates of the surface is taken along \(x_{1} - ,x_{2} - \,\,{\text{and}}\,\,x_{3} -\) directions with velocities \(u_{1} ,u_{2} \,\,{\text{and}}\,\,u_{3}\) respectively. The extended surface is placed along \(x_{1} -\) and \(x_{2} -\) directions with velocities \(u_{1w} = ax_{1}\) and \(u_{2w} = bx_{2}\) respectively where \(a\,\,and\,\,b\) are positive constants. The \(x_{3} -\) axis is normal to the \(\left( {x_{1} ,x_{2} } \right)\) plane i.e. perpendicular to the flow direction. The surface temperature and concentration of the sheet is denoted by \(T_{w} \,\,and\,\,C_{w}\) . Whereas the ambient temperature and concentration are \(T_{\infty } \,\,and\,\,C_{\infty }\) . A transversal magnetic field is applied parallel to \(x_{3} -\) axis with strength \(B_{0}\) . The thermal radiation and first-order chemical reaction are incorporated in energy and concentration equation. Additionally, the velocity slip, thermal slip, and concentration slip conditions are taken into consideration. The below equations describe the MHD three-dimensional hybrid nanofluid flow over a bi-directional stretching surface under the above-mentioned assumptions 39 , 40 :
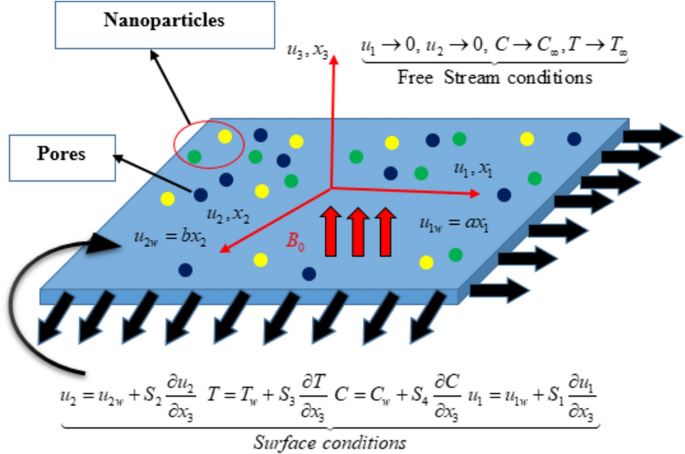
Geometry of flow problem.
The boundary conditions are given below 39 , 41 :
The similarity transformations are given as 40 ;
Here \(S_{1} ,S_{2} ,S_{3} \,and\,S_{4}\) are the velocities temperature and concentration slip parameters respectively. \(q_{a}\) is the Roseland approximation for radiative heat flux along \(x_{3} -\) direction and defined as below 26 , 42 :
Ignored the higher order term, the generalized form of Taylor series for \(T^{4}\) is:
Using Eq. ( 8 and 9 ) the Eq. ( 4 ) can be written as:
Employing the similarity transformation in Eq. ( 6 ) to the equations represented from serial (1 to 5) the nonlinear systems of ODEs are:
The transformed boundary conditions are:
\(Pr\left( { = \frac{{\vartheta_{f} \left( {\tilde{\rho }C_{p} } \right)_{f} }}{{\tilde{k}_{f} }}\,} \right)\) is the Prandtl number, \(Nt\left( { = \frac{{\left( {\tilde{\rho }C_{p} } \right)_{np} D_{T} \left( {T_{w} - T_{\infty } } \right)}}{{\left( {\tilde{\rho }C_{p} } \right)_{f} \vartheta_{f} T_{\infty } }}} \right)\) is thermophoresis parameter, \(N_{1} \left( { = S_{1} \sqrt {\frac{d}{{\vartheta_{f} }}} } \right)\) is the velocity slip parameter, \(Sc\left( { = \frac{{\vartheta_{f} }}{{D_{B} }}} \right)\) is Schmidt number, \(\beta \left( { = \frac{b}{d}} \right)\) is ratio parameter, \(K_{P} \left( { = \frac{{\mu_{f} }}{{\tilde{\rho }_{f} dk_{p} }}} \right)\) is porosity parameter, \(Rd\left( { = \frac{{4\dddot \sigma_{x} T_{\infty }^{3} }}{{k_{e} \tilde{k}_{f} }}} \right)\) is Radiation parameter, \(M\left( { = \frac{{\dddot \sigma_{f} B_{0}^{2} }}{{\tilde{\rho }_{f} d}}} \right)\) is the magnetic, \(N_{2} \left( { = S_{2} \sqrt {\frac{d}{{\vartheta_{f} }}} } \right)\) is the velocity slip parameter along y direction, \(Nb\left( { = \frac{{\left( {\tilde{\rho }C_{p} } \right)_{np} D_{B} \left( {C_{w} - C_{\infty } } \right)}}{{\left( {\tilde{\rho }C_{p} } \right)_{f} \delta_{c} \vartheta_{f} }}} \right)\) is Brownian motion parameter, \(N_{3} \left( { = S_{3} \sqrt {\frac{d}{{\vartheta_{f} }}} } \right)\) is thermal slip parameter, \(R_{x} = \frac{{k_{q} }}{d}\) is chemical reaction parameter, and solutal Slip factor is \(N_{4} \left( { = S_{4} \sqrt {\frac{d}{{\vartheta_{f} }}} } \right)\) . Here \(B_{1} = \frac{{\mu_{hnf} }}{{\mu_{f} }}\) , \(B_{2} = \frac{{\tilde{\rho }_{hnf} }}{{\tilde{\rho }_{f} }}\) , \(B_{3} = \frac{{\dddot \sigma_{hnf} }}{{\dddot \sigma_{f} }}\) , \(B_{4} = \frac{{\tilde{k}_{hnf} }}{{\tilde{k}_{f} }}\) and \(B_{5} = \frac{{\left( {\tilde{\rho }C_{p} } \right)_{hnf} }}{{\left( {\tilde{\rho }C_{p} } \right)_{f} }}\) are constants.
The theoretical model of hybrid nanofluid is given in (Table 1 ). In which \(\left( \mu \right)\) is the dynamic viscosity, the density is denoted by \(\left( {\tilde{\rho }} \right)\) , \(\left( {\tilde{\rho }C_{p} } \right)\) is the heat capacitance, \(\tilde{k}\) is the thermal conductivity, \(\left( {\dddot \sigma } \right)\) and is the electrical conductivity of the fluid. The subscript \(hnf,nf\,\,and\,\,f\) are used for hybrid, nanofluid, and base fluid respectively. \(\overset{\lower0.5em\hbox{$\smash{\scriptscriptstyle\frown}$}}{\chi }_{1} and\,\overset{\lower0.5em\hbox{$\smash{\scriptscriptstyle\frown}$}}{\chi }_{2}\) are the nanoparticles volume fraction of \(Cu\) and \(Al_{2} O_{3}\) nanoparticles. The thermophysical property of \(Cu\) , \(Al_{2} O_{3}\) and base fluid are defined in (Table 2 ).
Quantities of interest
The physical quantities which are important in engineering science and industrial process are 42 , 43 , 44 :
Using Eqs. ( 5 and 17 ) the simplified form of the Eq. ( 16 ) is given by:
where \(Nu_{x}\) represent Nusselt number, \(C_{{x_{1} }} \,\,\,{\text{and}}\,\,\,C_{{x_{2} }}\) are skin friction coefficients and \(S_{x}\) are mass transfer rate.
Numerical procedure
To solve the ODEs the bvp4c solver is used in MATLAB software. To set the problem for bvp4c the given below steps are as follows:
The boundary value problem is defined.
Apply the similarity transformations.
Achieved the nonlinear ODEs, with the boundary constraints.
Write the boundary value problem in first-order ordinary differential equation, so that we can easily compute it in MATLAB.
Find obtained the outcomes graphically and numerically.
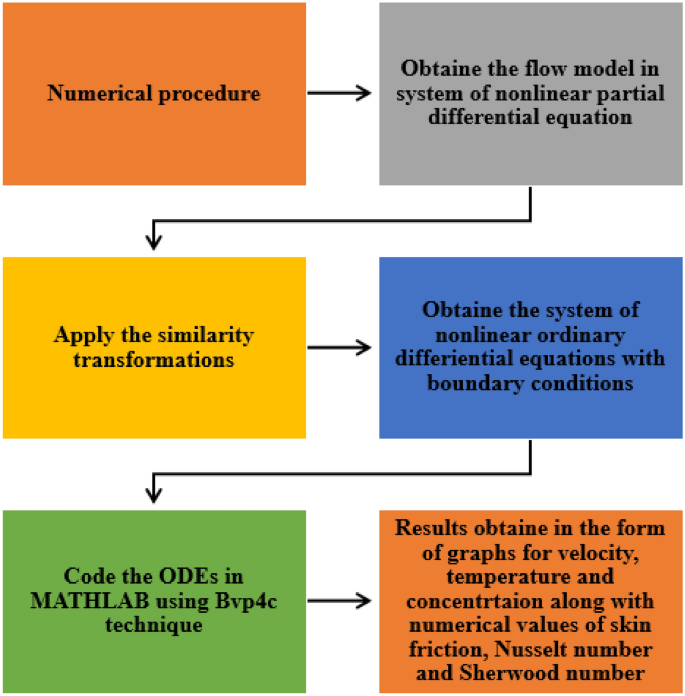
Using Eq. ( 20 ) we write the equation from (10–13) in the following form:
The new converted boundary circumstances are:
Validation of code
Comparison with previously published paper from the existing literature has been made to verify the precision of the present results. Table 2 demonstrates that the numerical values of the friction drag coefficients \(f^{\prime\prime}\left( 0 \right)\) and \(g^{\prime\prime}\left( 0 \right)\) in this paper across various \(\beta\) values closely align with the results published by Hayat et al. 38 . Consequently, we have confidence in the high accuracy of our results for analyzing the flow problem.
This section contain the impact of different physical parameters on MHD hybrid nanofluid slip flow over a bi-directional extended surface. These physical parameters are Magnetic parameter \(M\) , radiation parameter \(Rd\) velocity slip parameters \(N_{1} \,\,and\,\,N_{2}\) , concentration slip \(N_{3}\) , thermal slip parameters \(N_{4}\) , thermophoresis \(Nt\) , Brownian motion \(Nb\) and chemical reaction \(R_{x}\) . The flow fields are velocity along \(x -\) and \(y -\) directions, temperature and concentration represented by \(f^{\prime}\left( \xi \right)\) , \(g^{\prime}\left( \xi \right)\) , \(\theta \left( \xi \right)\) and \(\Phi \left( \xi \right)\) respectively. The skin frictions are \(C_{{x_{1} }} \,\,and\,\,C_{{x_{2} }}\) , Nusselt number \(N_{x}\) and mass transfer is \(S_{x}\) . Table 2 represents the thermophysical characteristics of the nanoparticles and base fluid. Table 3 shows the comparison of the present work with the already published work. This results shows that the current values are closely aligned with the work of Hayat et al. 38 . Figures 2 , 3 illustrates the effect of \(M\) on \(f^{\prime}\left( \xi \right)\) and \(g^{\prime}\left( \xi \right)\) velocities of the hybrid nanofluids respectively. An enhancement in \(M\) results in a decrease in the velocity profiles due to an external resistance. Consequently, the velocity gradients slow down leading to a reduction in the thickness of the momentum boundary layer and drop in the flow velocities. Physically the amplified value of \(M\) generates Lorentzian MHD drag force, which opposing fluid motion across the entire domain. Additionally the \(M\) combined with the slip effect functions as a hindering force. This retarding force can regulate the fluid velocity proving beneficial in various application such as coating of wire, metal and MHD power generation etc. In Figs. 4 , 5 the influence of the velocity slip parameters \(N_{1}\) and \(N_{2}\) on \(f^{\prime}\left( \xi \right)\) and \(g^{\prime}\left( \xi \right)\) are elucidate. Elevating \(N_{1}\) and \(N_{2}\) induces a reduction in \(f^{\prime}\left( \xi \right)\) and \(g^{\prime}\left( \xi \right)\) . Particularly the augmentation of \(N_{1}\) and \(N_{2}\) is associated with a reduction in the thickness of the momentum boundary layer, leading to a subsequent decrease in the velocity within this layer. Under slip conditions, it is noteworthy that the velocity of the stretching surface differs from that of the flow over the surface. In the given hybrid nanofluid flow over a linearly two directional extending surface both \(N_{1}\) and \(N_{2}\) exhibit identical effects along \(x_{1} -\) and \(x_{2} -\) directions as depicted in (Figs. 4 , 5 ). Figures 6 , 7 explore the impact of \(\beta\) on \(g^{\prime}\left( \xi \right)\) and \(f^{\prime}\left( \xi \right)\) . An increase in \(\beta\) manifests as a dual effect on \(g^{\prime}\left( \xi \right)\) and \(f^{\prime}\left( \xi \right)\) . Specifically a higher value of \(\beta\) correlates with a reduction in \(f^{\prime}\left( \xi \right)\) and a simultaneous increase in \(g^{\prime}\left( \xi \right)\) . The mathematical definition shows that \(\beta \left( { = \frac{b}{d}} \right)\) has inverse relation with the extending velocity constant \(d\) along \(x_{1} -\) direction. Conversely a directly relationship is observed with constant stretching velocity \(b\) along \(x_{2} -\) direction. Consequently the rise in \(\beta\) leads to a decrease in \(f^{\prime}\left( \xi \right)\) due to its inverse relation with \(d\) while concurrently augmenting \(g^{\prime}\left( \xi \right)\) owing to its direct relation with \(b\) as a result escalating \(\beta\) enhances \(g^{\prime}\left( \xi \right)\) and reduces \(f^{\prime}\left( \xi \right)\) of the hybrid nanofluid. Particles' average velocity decrease due to this enhanced rotation, which obstructs their linear translational motion. A more organized and constrained movement of the particles is produced by the interaction of rotational and translational dynamics, which helps to explain the decrease in \(f^{\prime}\left( \xi \right)\) . The sway of \(Nt\) on \(\theta \left( \xi \right)\) is display in (Fig. 8 ). This Figure shows that an elevation in \(Nt\) there is a corresponding increase in \(\theta \left( \xi \right)\) of hybrid nanofluid. This phenomenon is attributed to the heightened thermal resistance of the fluid driven by the tendency of thermophoretic distribution to elevate the non-dimensional fluid temperature. The catalyst behind this occurrence is the temperature gradient. As the \(Nt\) rise fluid particles undergo a movement toward higher energy levels, departing from colder regions. This migration contributes to an elevation in the \(\theta \left( \xi \right)\) profile. This is due to the fact that nanoparticles have the ability to carry heat more efficiently, which ultimately results in a more effective transfer of thermal energy throughout the fluid. Additionally, the increased motion of the nanoparticles can have the effect of enhancing the mixing of the nanofluid, which can also contribute to an increase in temperature. This shows a direct link of \(Nt\) with \(\theta \left( \xi \right)\) . The influence of \(Nb\) on \(\theta \left( \xi \right)\) is depicts in (Fig. 9 ). As the values of \(Nb\) enhance there is a corresponding growth in \(\theta \left( \xi \right)\) .This behavior can be elucidated by the fact that augmenting the \(Nb\) values results in heightened random motion among fluid particles. This escalated random motion in turn elevates the mean kinetic energy of fluid particles, leading to an increase in \(\theta \left( \xi \right)\) of hybrid nanofluid. Figure 10 display the impacts of the thermal slip parameter \(N_{3}\) on \(\theta \left( \xi \right)\) .The augmented values of \(N_{3}\) decline the curve of \(\theta \left( \xi \right)\) . Physically even with a modest amount of heat transferred from the sheet to the fluid the values of wall temperature and thermal boundary layer thickness decrease with an increase in \(N_{3}\) parameter. This implies that a higher \(N_{3}\) values induces a more efficient heat transfer mechanism resulting in a reduction of both wall temperature and thermal boundary layer thickness. As a result \(\theta \left( \xi \right)\) curves of hybrid nanofluid drops with the growing values of \(N_{3}\) . The impact of \(Rd\) on \(\theta \left( \xi \right)\) is illustrated in (Fig. 11 ). The finding shows that the \(\theta \left( \xi \right)\) rises with the expansion of \(Rd\) values. This relationship is explained by the fact that when \(Rd\) enrich the fluid receives more heat leading to an augmentation in both temperature and thermal boundary layer thickness. It is quite probable that the radiation factor denotes the system's radiative heat transfer mechanisms and their strength. The thermal equilibrium and redistribution of heat are affected by the increased interchange of thermal energy by electromagnetic waves, which occurs when the radiation factor increases. The radiative heat process signifies due to the augmentation in thermal distribution has a heightened impact on temperature. The change in \(\Phi \left( \xi \right)\) with the escalating value of \(N_{4}\) is shown in (Fig. 12 ). From Fig. 12 it is eminent that \(\Phi \left( \xi \right)\) decreases when \(N_{4}\) increases. The impact of \(R_{x}\) on \(\Phi \left( \xi \right)\) is depicts in (Fig. 13 ). The \(\Phi \left( \xi \right)\) panel diminishes as \(R_{x}\) parameter enhances. This occurrence is attributed to the heightened participation of particles in the chemical reaction resulting in an over reduction in the concentration profile. Physically when \(R_{x}\) grows, the quantity of solute molecules increases, resulting in a drop in \(\Phi \left( \xi \right)\) . The thickness of the solutal boundary layer is significantly reduced by destructive chemical reactions. Therefore the enrichment in \(R_{x}\) , decline the \(\Phi \left( \xi \right)\) of hybrid nanofluid. The impact of \(Nt\) on \(\Phi \left( \xi \right)\) is portraits in (Fig. 14 ). The impact of \(Nt\) on \(\Phi \left( \xi \right)\) is characterized by a monotonic trend, as the values of \(Nt\) grows the concentration boundary layer thickness also upsurges. Furthermore the graph illustrates the magnitude of the concentration gradient on the sheets surface diminishes with higher values of \(Nt\) . Additional the Sherwood number \(S_{x} \left( { = - \Phi^{\prime}\left( 0 \right)} \right)\) which signifies the mass transfer rate at the surface decreases as \(Nt\) increases is shown is (Table 6 ). This decrease more pronounced in hybid nanofluid as compared to nanofluid. Thermophoresis describes the movement of particles driven by temperature gradient. As the thermophoresis factors enhance it exerts a stronger effect on the distribution of thermal energy and particle concentrations. This increase indicates that the system undergoes a more significant separation of particles according to their thermal responses. The enhanced thermophoresis factor impacts the thermal distribution by causing particles to move in the temperature gradient direction, thereby altering temperature. At the same time, it affects the concentration distribution by causing particles to migrate based on their size shape, and thermal properties. The change in \(\Phi \left( \xi \right)\) in response to varying \(Nb\) is shown in (Fig. 15 ). As upsurge in \(Nb\) results in a reduction of the concentration boundary thickness. Additionally the concentration gradient magnitude at the sheets surface rises with the higher values of \(Nb\) . Consequently \(S_{x} \left( { = - \Phi^{\prime}\left( 0 \right)} \right)\) of the hybrid nanofluid at the surface enhances with the hike of \(Nb\) shown in (Table 6 ). The random movement of nanoparticles in this phenomena results in a reduction in their kinetic energy, which in turn results in a reduction in the rate at which heat is transmitted. As the value of Nb grows, the number of collisions between fluid particles also increases, which results in a decrease in the amount of mass that is transferred from the heated sheet to the cool fluid. Figures 16 , 17 shows the impacts of \(M\) on the streams lines and countours plots. From these Figures it is clear that with the higher values of \(M\) the streams lines expands while the distance between the curves of contours lines suppress . This demonstrates that the streamline pattern has been altered as a result of the increased intensity of the magnetic field. Table 4 illustrates the change in the \(f^{\prime\prime}\left( 0 \right)\) and \(g^{\prime\prime}\left( 0 \right)\) concerning with no slip \(N_{1} = N_{2} = 0\) and slip \(N_{1} = N_{2} = 0.2\) effects. Upon examining the Table 4 it becomes evident that with an increase in \(M\) there is a corresponding increase in the values of \(f^{\prime\prime}\left( 0 \right)\) and \(g^{\prime\prime}\left( 0 \right)\) . Furthermore in the presence of slip effect the skin friction is reduces. The effect of \(Nt,Nb,Rd\,\,and\,\,N_{3}\) on Nusselt number are shown in (Table 5 ). The ascending values of \(Nt,Nb\,\,and\,\,N_{3}\) parameters diminishes the heat transfer rate whereas the \(Rd\) enhancements the Nusselt number. The effect of \(Nt,Nb,R_{x} \,\,and\,\,N_{4}\) on Sherwood number are shown in (Table 6 ). As the growing values of \(Nt\,\,and\,\,N_{4}\) parameters reduce the mass transfer rate whereas the \(R_{x} \,\) and \(,Nb\) boosts the Sherwood number.
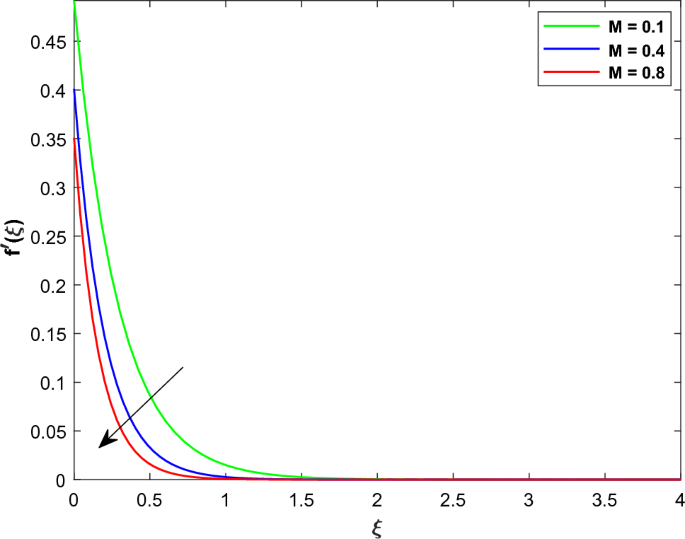
\(f^{\prime}\left( \xi \right)\) profile for various values of \(M\) .
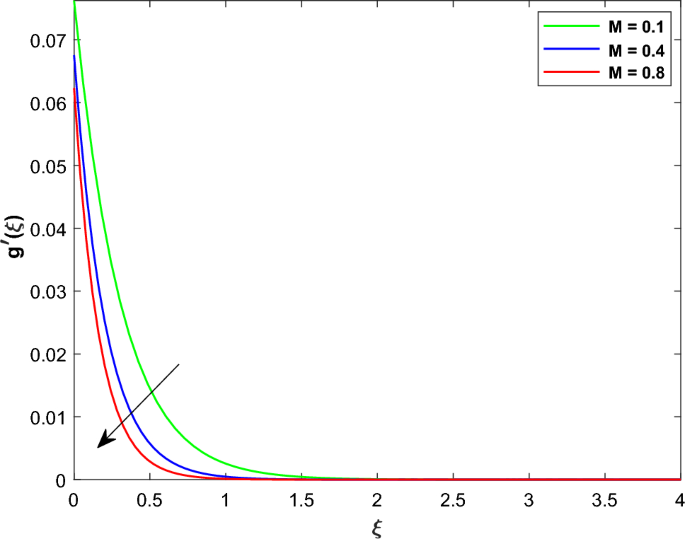
\(g^{\prime}\left( \xi \right)\) profile for various values of \(M\) .
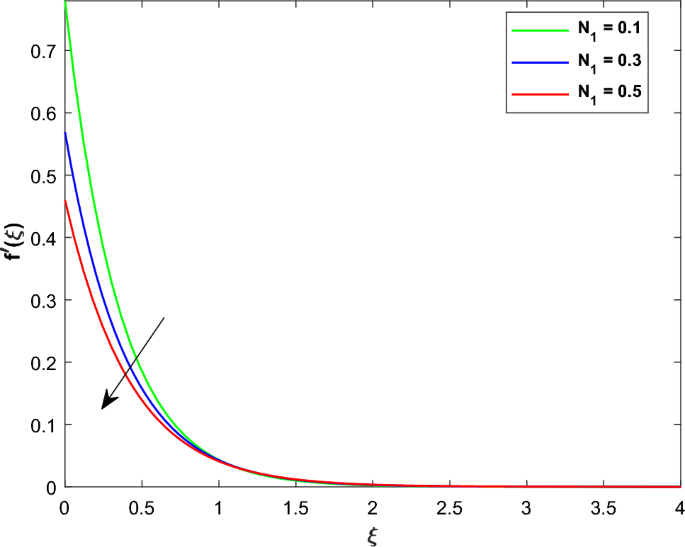
\(f^{\prime}\left( \xi \right)\) profile for various values of \(N_{1}\) .
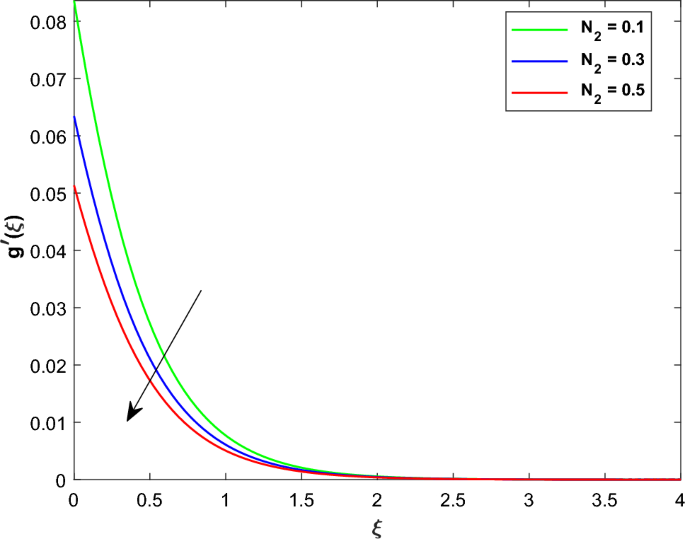
\(g^{\prime}\left( \xi \right)\) profile for various values of \(N_{2}\) .
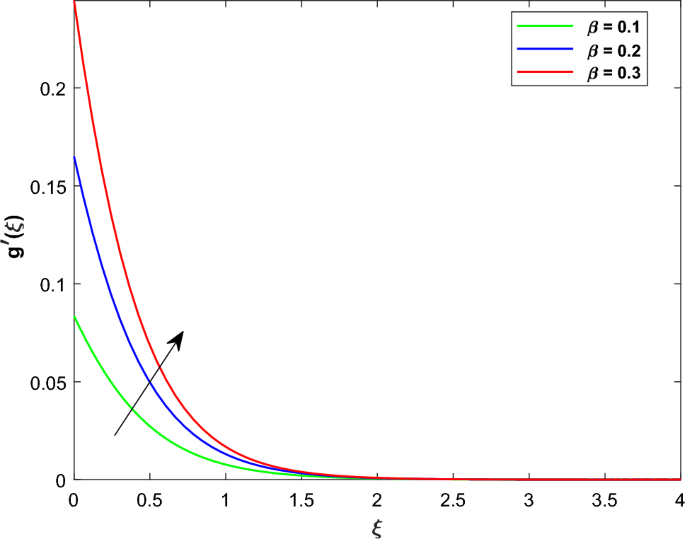
\(g^{\prime}\left( \xi \right)\) profile for various values of \(\beta\) .

\(f^{\prime}\left( \xi \right)\) profile for various values of \(\beta\) .
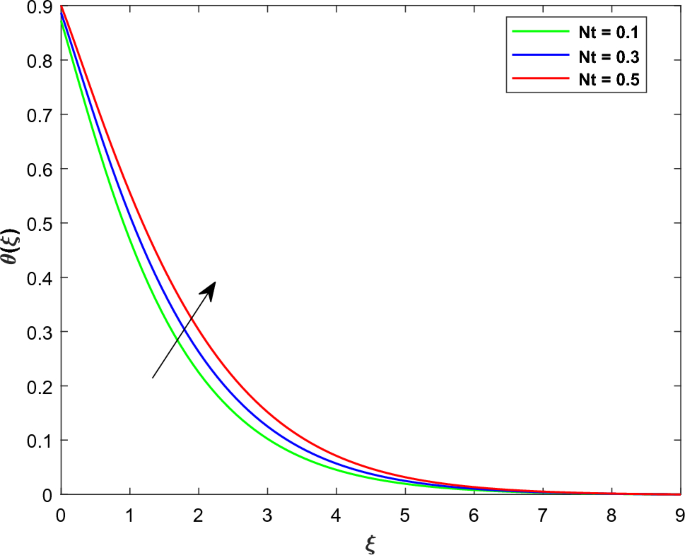
\(\theta \left( \xi \right)\) profile for various values of \(Nt\) .
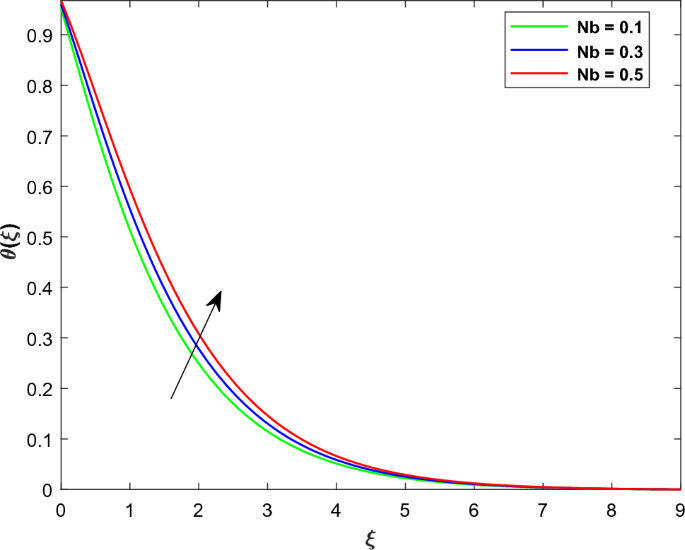
\(\theta \left( \xi \right)\) profile for various values of \(Nb\) .

\(\theta \left( \xi \right)\) profile for various values of \(N_{3}\) .

\(\theta \left( \xi \right)\) profile for various values of \(Rd\) .
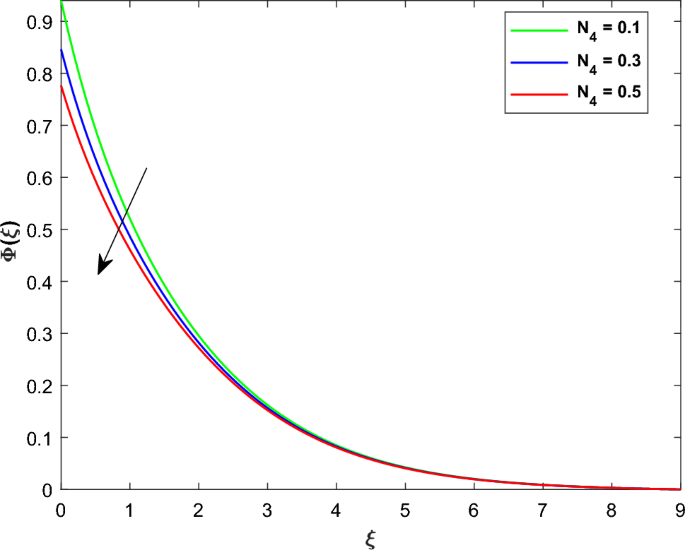
\(\Phi \left( \xi \right)\) profile for various values of \(N_{4}\) .

\(\Phi \left( \xi \right)\) profile for various values of \(R_{x}\) .

\(\Phi \left( \xi \right)\) profile for various values of \(Nt\) .

\(\Phi \left( \xi \right)\) profile for various values of \(Nb\) .

The stream lines and contours plots for for \(f\left( \eta \right)\) . ( a ) For \(M = 0.1\) , ( b ) For \(M = 0.5\) , ( c ) For \(M = 0.1\) , ( d ) For \(M = 0.5\) .
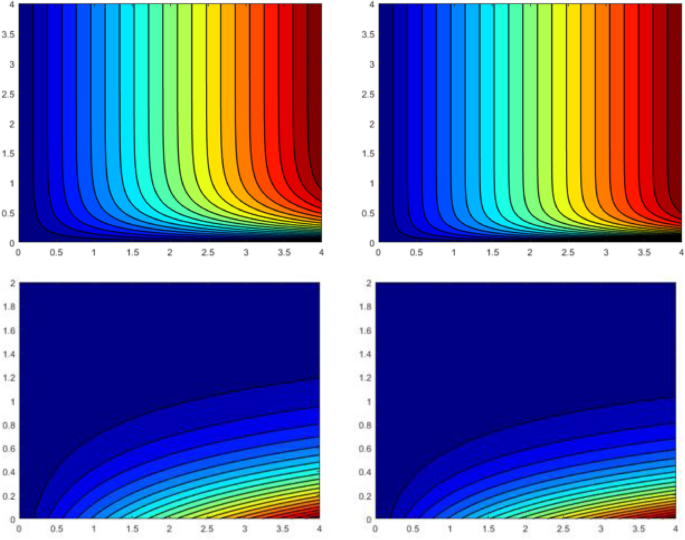
The stream lines and contours plots for \(g\left( \eta \right)\) .
Final remarks
There has been a revolution in the field of thermal engineering as a result of the improved potential of hybrid nanofluids, which significantly increases the efficiency of heat exchangers and saves energy. The current declaration intends to inspect a numerical analysis of the heat and mass transfer characteristics of three dimensional MHD hybrid nanofluid flow over a porous bi-directional stretching sheet, considering slip effects. The flow is assumed to be laminar, steady, and incompressible. A hybrid nanofluid has been composed by dispersing of Cu and Al 2 O 3 nanoparticles in water. The governing equations initially formulated as partial differential equations and then transformed into a nonlinear system of ordinary differential equations through appropriate transformations. The bvp4c technique in MATLAB is employed for solving these equations. Several involve parameters impacts on flow distributions have been studied and elucidated through graphs and tables. The slip conditions are also used to examine different distributions of flows. The accuracy of the numerical approach is validated by comparing the results with previously published work demonstrating excellent agreement. The study's findings are summarized as follows:
The fluid velocity along \(x_{1} -\) and \(x_{2} -\) directions slowed down with the rising values of the magnetic and slip parameters.
The ratio parameter has dual impact on velocity along \(x_{1} -\) and \(x_{2} -\) directions. The amplified value of beta enhance the velocity along \(x_{2} -\) direction and suppress along \(x_{1} -\) direction.
The temperature profile grows with radiation and thermophoresis parameters.
An elevation in the thermal slip parameter led to a reduction in both the boundary layer thickness and the heat transfer from the surface to the fluid.
The concentration profiles exhibits increment with thermophoresis parameter while decline with rising chemical reaction, Brownian motion parameters.
Growing values of the concentration slip parameter reduced the mass transfer resulting in a reduction of the concentration profile.
The higher values of magnetic parameter decrease the skin friction coefficient for both slip and no slip. The skin friction is higher for no slip condition.
The heat transfer rate decreasing function of Brownian, thermophoresis while increasing with radiation factor.
The mass transfer rate boosts with Brownian and chemical reaction and declines with thermophoresis parameter.
The stream lines becomes wide with the improved values of magnetic parameter while the contours plots close to each other.
Future direction
In Future work, we can focus on the effect of various factors such as:
Variable chemical reaction of higher order.
Activation energy.
Nonlinear thermal radiation and heat source.
Different types of nanoparticles.
Data availability
The datasets used and/or analysed during the current study available from the corresponding author on reasonable request.
Abbreviations
Porosity parameter \(\left[ - \right]\)
Velocity along \(x_{1} - ,x_{2} - \,\,and\,x_{3} -\) axis \(\left[ {ms^{ - 1} } \right]\)
Coordinates of axis
Velocity slip parameter along \(x -\) and \(y -\) directions \(\left[ - \right]\)
Stretching velocities \(\left[ {ms^{ - 1} } \right]\)
Thermophoresis parameter \(\left[ - \right]\)
Ratio parameter \(\left[ - \right]\)
Chemical reaction \(\left[ - \right]\)
Radiation parameter \(\left[ - \right]\)
Brownian motion \(\left[ - \right]\)
Thermal slip parameter \(\left[ - \right]\)
Dynamic viscosity \(\left[ {Kgm^{ - 1} S^{ - 1} } \right]\)
Thermal conductivity \(\left[ {Wm^{ - 1} K} \right]\)
Strength of the magnetic field \(\left[ {kgS^{ - 2} A^{ - 1} } \right]\)
Temperature of the fluid \(\left[ K \right]\)
Prandtl number \(\left[ - \right]\)
Brownian diffusion coefficient \(\left[ {m^{2} s^{ - 1} } \right]\)
Ambient temperature \(\left[ K \right]\)
Concentration slip parameter
Thermophoreis diffusion coefficient \(\left[ {m^{2} s^{ - 1} } \right]\)
Wall temperature \(\left[ K \right]\)
Ambient concentration \(\left[ - \right]\)
Concentration of the fluid \(\left[ - \right]\)
Skin friction coefficient \(x_{1} - \,\,and\,\,x_{2} -\) directions \(\left[ - \right]\)
Local Nusselt number \(\left[ - \right]\)
Positive constants along \(x_{1} - \,\,and\,\,x_{2} -\) directions \(\left[ - \right]\)
Specific heat \(\left[ {Jkg^{ - 1} K^{ - 1} } \right]\)
Sherwood number \(\left[ - \right]\)
Stefan-Boltzmann constant \(\left[ {Wm^{ - 2} K^{ - 4} } \right]\)
Schmidt number \(\left[ - \right]\)
Radiative heat flux \(\left[ {Wm^{ - 2} } \right]\)
Electrical conductivity \(\left[ {\Omega^{ - 1} m^{ - 1} } \right]\)
Surface concentration \(\left[ - \right]\)
Density \(\left[ {Kgm^{ - 3} } \right]\)
Base fluid \(\left[ - \right]\)
- Hybrid nanofluid
Choi, S.U.S. & Eastman, J.A. Enhancing Thermal Conductivity of Fluids with Nanoparticles . (ASME International Mechanical Engineering Congress & Exposition, San Francisco, 1995).
Choi, S. U. S., Zhang, Z. G., Yu, Wl., Lockwood, F. E. & Grulke, E. A. Anomalous thermal conductivity enhancement in nanotube suspensions. Appl. Phys. Lett. 79 , 2252–2254 (2001).
Article ADS CAS Google Scholar
Vedavathi, N., Dharmaiah, G., Venkatadri, K. & Gaffar, S. A. Numerical study of radiative non-darcy nanofluid flow over a stretching sheet with a convective nield conditions and energy activation. Nonlinear Eng. 10 , 159–176 (2021).
Article ADS Google Scholar
Abas, S. A., Ullah, H., Islam, S. & Fiza, M. A passive control of magnetohydrodynamic flow of a blood-based Casson hybrid nanofluid over a convectively heated bi-directional stretching surface. Z Angew. Math Mech. 104 , e202200576 (2023).
Article MathSciNet Google Scholar
Ali, F., Zaib, A., Reddy, S., Alshehri, M. H. & Shah, N. A. Impact of thermal radiative carreau ternary hybrid nanofluid dynamics in solar aircraft with entropy generation: Significance of energy in solar aircraft. J. Therm. Anal. Calorim. 149 , 1495–1513 (2024).
Article CAS Google Scholar
Saeed, A. et al. Darcy-Forchheimer hybrid nanofluid flow over a stretching curved surface with heat and mass transfer. PLoS ONE 16 , e0249434 (2021).
Article CAS PubMed PubMed Central Google Scholar
Guedri, K. et al. Thermally dissipative flow and entropy analysis for electromagnetic trihybrid nanofluid flow past a stretching surface. ACS Omega 7 , 33432–33442 (2022).
Dawar, A., Shah, Z., Alshehri, H. M., Islam, S. & Kumam, P. Magnetized and non-magnetized Casson fluid flow with gyrotactic microorganisms over a stratified stretching cylinder. Sci. Rep. 11 , 16376 (2021).
Article ADS CAS PubMed PubMed Central Google Scholar
Zangooee, M. R., Hosseinzadeh, K. & Ganj, D. D. Investigation of three-dimensional hybrid nanofluid flow affected by nonuniform MHD over exponential stretching/shrinking plate. Nonlinear Eng. 11 , 143–155 (2022).
Rehman, A., Khan, D., Mahariq, I., Elkotb, M. A. & Elnaqeeb, T. Viscous dissipation effects on time-dependent MHD Casson nanofluid over stretching surface: A hybrid nanofluid study. J. Mol. Liq. 408 , 125370 (2024).
Crane, L. J. Flow past a stretching plate. Zeitschrift für Angew. Math. Phys. ZAMP 21 , 645–647 (1970).
Shamshuddin, M. D., Saeed, A., Mishra, S. R., Katta, R. & Eid, M. R. Homotopic simulation of MHD bioconvective flow of water-based hybrid nanofluid over a thermal convective exponential stretching surface. Int. J. Numer. Methods Heat Fluid Flow 34 , 31–53 (2024).
Article Google Scholar
Das, K. Nanofluid flow over a non-linear permeable stretching sheet with partial slip. J. Egypt. Math. Soc. 23 , 451–456 (2015).
Alarifi, I. M. et al. MHD flow and heat transfer over vertical stretching sheet with heat sink or source effect. Symmetry 11 , 297 (2019).
Waqas, H. et al. Heat transfer analysis of hybrid nanofluid flow with thermal radiation through a stretching sheet: A comparative study. Int. Commun. Heat Mass Transf. 138 , 106303 (2022).
Shamshuddin, M. D., Asogwa, K. K. & Ferdows, M. Thermo-solutal migrating heat producing and radiative Casson nanofluid flow via bidirectional stretching surface in the presence of bilateral reactions. Numer. Heat Transf. Part A Appl. 85 , 719–738 (2024).
Bouslimi, J. et al. MHD Williamson nanofluid flow over a stretching sheet through a porous medium under effects of joule heating, nonlinear thermal radiation, heat generation/absorption, and chemical reaction. Adv. Math. Phys. 2021 , 1–16 (2021).
Lone, S. A. et al. Computational analysis of MHD driven bioconvective flow of hybrid Casson nanofluid past a permeable exponential stretching sheet with thermophoresis and Brownian motion effects. J. Magn. Magn. Mater. 580 , 170959 (2023).
Khan, U., Waini, I., Ishak, A. & Pop, I. Unsteady hybrid nanofluid flow over a radially permeable shrinking/stretching surface. J. Mol. Liq. 331 , 115752 (2021).
Jamshed, W., Devi, S. U. & Nisar, K. S. Single phase based study of Ag-Cu/EO Williamson hybrid nanofluid flow over a stretching surface with shape factor. Phys. Scr. 96 , 065202 (2021).
Abbas, N., Rehman, K. U., Shatanawi, W. & Malik, M. Y. Numerical study of heat transfer in hybrid nanofluid flow over permeable nonlinear stretching curved surface with thermal slip. Int. Commun. Heat Mass Transf. 135 , 106107 (2022).
Sajid, M., Ali, N., Abbas, Z. & Javed, T. Flow of a micropolar fluid over a curved stretching surface. J. Eng. Phys. Thermophys. 84 , 864–871 (2011).
Kumar, M. A., Reddy, Y. D., Goud, B. S. & Rao, V. S. Effects of soret, dufour, hall current and rotation on MHD natural convective heat and mass transfer flow past an accelerated vertical plate through a porous medium. Int. J. Thermofluids 9 , 100061 (2021).
Reddy, M. V. et al. Magneto-Williamson nanofluid flow past a wedge with activation energy: Buongiorno model. Adv. Mech. Eng. 16 , 16878132231223028 (2024).
Ali, A. et al. Hall effects and Cattaneo-Christov heat flux on MHD flow of hybrid nanofluid over a varying thickness stretching surface. Mod. Phys. Lett. B 38 , 2450130 (2024).
Vishalakshi, A. B. et al. MHD hybrid nanofluid flow over a stretching/shrinking sheet with skin friction: Effects of radiation and mass transpiration. Magnetochemistry 9 , 118 (2023).
Naveed, M., Abbas, Z. & Sajid, M. Hydromagnetic flow over an unsteady curved stretching surface. Eng. Sci. Technol. Int. J. 19 , 841–845 (2016).
Google Scholar
Andersson, H. I. Slip flow past a stretching surface. Acta Mech. 158 , 121–125 (2002).
Wang, C. Y. Flow due to a stretching boundary with partial slip—An exact solution of the Navier-Stokes equations. Chem. Eng. Sci. 57 , 3745–3747 (2002).
Hayat, T., Qasim, M. & Mesloub, S. MHD flow and heat transfer over permeable stretching sheet with slip conditions. Int. J. Numer. Methods Fluids 66 , 963–975 (2011).
Article ADS MathSciNet Google Scholar
Sharma, S. et al. MHD micro polar fluid flow over a stretching surface with melting and slip effect. Sci. Rep. 13 , 10715 (2023).
Ali, F. et al. Scrutinization using both numerical and analytical techniques for darcy forchheimer flow in the gyrotactic microorganism of nanofluid over a rotating disk. Numer. Heat Transf. Part B Fundam. https://doi.org/10.1080/10407790.2023.2300678 (2024).
Raza, J., Rohni, A. M., Omar, Z. & Awais, M. Heat and mass transfer analysis of MHD nanofluid flow in a rotating channel with slip effects. J. Mol. Liq. 219 , 703–708 (2016).
Ramzan, M. et al. Heat transfer analysis of the mixed convective flow of magnetohydrodynamic hybrid nanofluid past a stretching sheet with velocity and thermal slip conditions. PLoS ONE 16 , e0260854 (2021).
Ali, B. et al. Numerical investigation of heat source induced thermal slip effect on trihybrid nanofluid flow over a stretching surface. Results Eng. 20 , 101536 (2023).
Syahirah Wahid, N. et al. Three-dimensional stretching/shrinking flow of hybrid nanofluid with slips and joule heating. J. Thermophys. Heat Transf. 36 , 848–857 (2022).
Anuar, N. S., Bachok, N. & Pop, I. Numerical computation of dusty hybrid nanofluid flow and heat transfer over a deformable sheet with slip effect. Mathematics 9 , 643 (2021).
Hayat, T., Awais, M. & Obaidat, S. Three-dimensional flow of a Jeffery fluid over a linearly stretching sheet. Commun. Nonlinear Sci. Numer. Simul. 17 , 699–707 (2012).
Razi, S. M., Soid, S. K., Abd Aziz, A. S., Adli, N. & Ali, Z. M. Williamson nanofluid flow over a stretching sheet with varied wall thickness and slip effects. J. Phys. Conf. Ser. 1366 , 012007 (2019).
Khan, I., Rahman, A. U., Dawar, A., Islam, S. & Zaman, A. Second-order slip flow of a magnetohydrodynamic hybrid nanofluid past a bi-directional stretching surface with thermal convective and zero mass flux conditions. Adv. Mech. Eng. 15 , 1–12 (2023).
Ibrahim, W. & Shankar, B. MHD boundary layer flow and heat transfer of a nanofluid past a permeable stretching sheet with velocity, thermal and solutal slip boundary conditions. Comput. Fluids 75 , 1–10 (2013).
Zainal, N. A., Nazar, R., Naganthran, K. & Pop, I. The impact of thermal radiation on Maxwell hybrid nanofluids in the stagnation region. Nanomaterials 12 , 1109 (2022).
Teh, Y. Y. & Ashgar, A. Three dimensional MHD hybrid nanofluid flow with rotating stretching/shrinking sheet and Joule heating. CFD Lett. 13 , 1–19 (2021).
Arshad, M. et al. Rotating hybrid nanofluid flow with chemical reaction and thermal radiation between parallel plates. Nanomaterials 12 , 4177 (2022).
Aly, E. H. & Pop, I. MHD flow and heat transfer near stagnation point over a stretching/shrinking surface with partial slip and viscous dissipation: Hybrid nanofluid versus nanofluid. Powder Technol. 367 , 192–205 (2020).
Khan, M., Ali, W. & Ahmed, J. A hybrid approach to study the influence of hall current in radiative nanofluid flow over a rotating disk. Appl. Nanosci. 10 , 5167–5177 (2020).
Download references
Author information
Authors and affiliations.
Department of Mathematics, Abdul Wali Khan University, Mardan, 23200, Khyber Pakhtunkhwa, Pakistan
Hakeem Ullah, Syed Arshad Abas & Mehreen Fiza
Department of Mathematics, Saveetha School of Engineering, SIMATS, Chennai, Tamil Nadu, India
Department of Mathematics, College of Science Al-Zulf, Majmaah University, Al-Majmaah, 11952, Saudi Arabia
Hourani Center for Applied Scientific Research, Al-Ahliyya Amman University, Amman, Jordan
Al-Ayen Research Center, Al-Ayen University, Nasiriyah, Iraq
Department of Mathematics, Education Faculty, Laghman University, Mehtarlam City, Laghman, 2701, Afghanistan
Ariana Abdul Rahimzai
Department of Computer Science and Mathematics, Lebanese American University, Beirut, Lebanon
Art and Science Faculty, Department of Mathematics, Siirt University, 56100, Siirt, Turkey
You can also search for this author in PubMed Google Scholar
Contributions
Hakeem Ullah (1), Syed Arshad Abas (2), Mehreen Fiza(3), Ilyas Khan(4), Ariana Abdul Rahimzai(5), Ali Akgul(6) Author’s Contribution authors (1,2,3,4,5,6) Conceptualization, Formal analysis, Resources, Software, Supervision, Writing—original draft authors (1,2,4,5,6) Methodology, Resources, Software, Validation, Writing—original draft authors (4,3,1,6) Resources, Validation, Writing—original draft authors (2,3,4,6) Resources, Visualization, authors (4,2,5,6) Resources, Validation, authors (1,5,3,2,4) Conceptualization, Formal analysis, Supervision, Visualization.
Corresponding author
Correspondence to Ariana Abdul Rahimzai .
Ethics declarations
Competing interests.
The authors declare no competing interests.
Additional information
Publisher's note.
Springer Nature remains neutral with regard to jurisdictional claims in published maps and institutional affiliations.
Rights and permissions
Open Access This article is licensed under a Creative Commons Attribution-NonCommercial-NoDerivatives 4.0 International License, which permits any non-commercial use, sharing, distribution and reproduction in any medium or format, as long as you give appropriate credit to the original author(s) and the source, provide a link to the Creative Commons licence, and indicate if you modified the licensed material. You do not have permission under this licence to share adapted material derived from this article or parts of it. The images or other third party material in this article are included in the article’s Creative Commons licence, unless indicated otherwise in a credit line to the material. If material is not included in the article’s Creative Commons licence and your intended use is not permitted by statutory regulation or exceeds the permitted use, you will need to obtain permission directly from the copyright holder. To view a copy of this licence, visit http://creativecommons.org/licenses/by-nc-nd/4.0/ .
Reprints and permissions
About this article
Cite this article.
Ullah, H., Abas, S.A., Fiza, M. et al. A numerical study of heat and mass transfer characteristic of three-dimensional thermally radiated bi-directional slip flow over a permeable stretching surface. Sci Rep 14 , 19842 (2024). https://doi.org/10.1038/s41598-024-70167-2
Download citation
Received : 29 February 2024
Accepted : 13 August 2024
Published : 27 August 2024
DOI : https://doi.org/10.1038/s41598-024-70167-2
Share this article
Anyone you share the following link with will be able to read this content:
Sorry, a shareable link is not currently available for this article.
Provided by the Springer Nature SharedIt content-sharing initiative
- Stretching surface
- Slip conditions and magnetohydrodynamic (MHD)
By submitting a comment you agree to abide by our Terms and Community Guidelines . If you find something abusive or that does not comply with our terms or guidelines please flag it as inappropriate.
Quick links
- Explore articles by subject
- Guide to authors
- Editorial policies
Sign up for the Nature Briefing: AI and Robotics newsletter — what matters in AI and robotics research, free to your inbox weekly.

Advertisement
Microstructure-based modelling of tensile properties of heat treated Inconel 718 alloy produced by laser powder bed fusion
- Original Article
- Published: 05 September 2024
- Volume 24 , article number 227 , ( 2024 )
Cite this article
- P. Kumnaknoppakun 1 &
- V. Uthaisangsuk ORCID: orcid.org/0000-0002-9070-2043 1
Due to the instability of the γ ″ phase at elevated temperatures, leading to the δ phase transformation, effects of the delta ( δ ) process treatment (DPT) on tensile stress–strain characteristics of Inconel 718 alloy fabricated by laser powder bed fusion (L-PBF) process at varying powers were investigated using experiments and microstructure-based modelling in this study. Additively manufactured test specimens were firstly subjected to a direct aging treatment and then followed by the DPT processes. Microstructure and fractography analyses of the heat treated samples were conducted and effects of δ phase emergence on resulting tensile properties were investigated. In addition, a microstructure-based approach using representative volume elements (RVEs) were proposed for describing stress–strain behaviors of specimens from different conditions. Observed microstructure features were incorporated and micromechanics parameters of the matrix and δ phase were determined. It was found that predicted flow stress curves fairly agreed with those from the experiments. Increasing amount and size of the δ phase in the printed Inconel samples by longer DPT holding time led to decreased strength due to the depletion of strengthening γ′′ phase, but enhanced strain hardening because of the pinning effect on dislocation migration by δ phase particles. Short-rod bulky δ phase at grain boundaries rather promoted earlier crack initiation and caused lowered fracture elongation than needle-like δ phase within the matrix. For built samples from conditions close to the border of process window, predictions of crack formation and total elongations by RVE simulations required accurately measured porosities and their interaction with the δ phase.
This is a preview of subscription content, log in via an institution to check access.
Access this article
Subscribe and save.
- Get 10 units per month
- Download Article/Chapter or eBook
- 1 Unit = 1 Article or 1 Chapter
- Cancel anytime
Price includes VAT (Russian Federation)
Instant access to the full article PDF.
Rent this article via DeepDyve
Institutional subscriptions
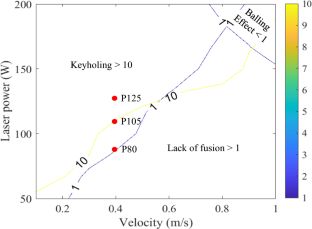
Data availability
The raw/processed data required to reproduce these findings cannot be shared at this time due to technical or time limitations.
Wang X, Gong X, Chou K. Review on powder-bed laser additive manufacturing of Inconel 718 parts. Proc Inst Mech Eng B J Eng Manuf. 2017;231(11):1890–903. https://doi.org/10.1177/0954405415619883 .
Article Google Scholar
Tucho WM, Cuvillier P, Sjolyst-Kverneland A, Hansen V. Microstructure and hardness studies of Inconel 718 manufactured by selective laser melting before and after solution heat treatment. Mater Sci Eng A. 2017;689:220–32. https://doi.org/10.1016/j.msea.2017.02.062 .
Ling LSB, Yin Z, Hu Z, Liang JH, Wang ZY, Wang J, Sun BD. Effects of the γ″-Ni3Nb phase on mechanical properties of Inconel 718 superalloys with different heat treatments. Materials. 2019;13:151. https://doi.org/10.3390/ma13010151 .
Gallmeyer TG, Moorthy S, Kappes BB, Mills MJ, Amin-Ahmadi B, Stebner AP. Knowledge of process-structure-property relationships to engineer better heat treatments for laser powder bed fusion additive manufactured Inconel 718. Addit Manuf. 2020;31:100977. https://doi.org/10.1016/j.addma.2019.100977 .
Gao Y, Zhang D, Cao M, Chen R, Feng Z, Poprawe R, Schleifenbaum JH, Ziegler S. Effect of δ phase on high temperature mechanical performances of Inconel 718 fabricated with SLM process. Mater Sci Eng A. 2019;767:138327. https://doi.org/10.1016/j.msea.2019.138327 .
Diepold B, Vorlaufer N, Neumeier S, Gartner T, Göken M. Optimization of the heat treatment of additively manufactured Ni-base superalloy IN718. Int J Miner Metall Mater. 2020;27:640–8. https://doi.org/10.1007/s12613-020-1991-6 .
Mohd Yusuf S, Cutler S, Gao N. Review: the impact of metal additive manufacturing on the aerospace industry. Metals. 2019;9:1286. https://doi.org/10.3390/met9121286 .
Aslan B, Yıldız AR. Optimum design of automobile components using lattice structures for additive manufacturing, Mater. TEST. 2020;62:633–9. https://doi.org/10.3139/120.111527 .
Kopar M, Yildiz AR. Experimental investigation of mechanical properties of PLA, ABS, and PETG 3-d printing materials using fused deposition modeling technique, Mater. TEST. 2023;65:1795–804. https://doi.org/10.1515/mt-2023-0202 .
Günaydın AC, Yıldız AR, Kaya N. Multi-objective optimization of build orientation considering support structure volume and build time in laser powder bed fusion, Mater. TEST. 2022;64:323–38. https://doi.org/10.1515/mt-2021-2075 .
Xu Y, Gong Y, Li P, Yang Y, Qi Y. The effect of laser power on the microstructure and wear performance of IN718 superalloy fabricated by laser additive manufacturing. Int J Adv Manuf Technol. 2020;108:2245–54. https://doi.org/10.1007/s00170-020-05172-6 .
Wang W, Wang S, Zhang X, Chen F, Xu Y, Tian Y. Process parameter optimization for selective laser melting of Inconel 718 superalloy and the effects of subsequent heat treatment on the microstructural evolution and mechanical properties. J Manuf Proc. 2021;64:530–43. https://doi.org/10.1016/j.jmapro.2021.02.004 .
Promoppatum P, Yao SC. Analytical evaluation of defect generation for selective laser melting of metals. Int J Adv Manuf Technol. 2019;103:1185–98. https://doi.org/10.1007/s00170-019-03500-z .
Pan H, Dahmen T, Bayat M, Lin K, Zhang X. Independent effects of laser power and scanning speed on IN718’s precipitation and mechanical properties produced by LBPF plus heat treatment. Mater Sci Eng A. 2022;849:143530. https://doi.org/10.1016/j.msea.2022.143530 .
Yuan K, Guo W, Li D, Li P, Zhang Y, Wang P. Influence of heat treatments on plastic flow of laser deposited Inconel 718: testing and microstructural based constitutive modeling. Int J Plast. 2021;136:102865. https://doi.org/10.1016/j.ijplas.2020.102865 .
Fayed EM, Saadati M, Shahriari D, Brailovski V, Jahazi M, Medraj M. Effect of homogenization and solution treatments time on the elevated-temperature mechanical behavior of Inconel 718 fabricated by laser powder bed fusion. Sci Rep. 2021;11:2020. https://doi.org/10.1038/s41598-021-81618-5 .
Zhang SH, Zhang HY, Cheng M. Tensile deformation and fracture characteristics of delta-processed Inconel 718 alloy at elevated temperature. Mater Sci Eng A. 2011;528:6253–8. https://doi.org/10.1016/j.msea.2011.04.074 .
Zhang XC, Li HC, Zeng X, Tu ST, Zhang C-C, Wang QQ. Fatigue behavior and bilinear Coffin-Manson plots of Ni-based GH4169 alloy with different volume fractions of δ phase. Mater Sci Eng A. 2017;682:12–22. https://doi.org/10.1016/j.msea.2016.11.040 .
Anderson M, Thielin AL, Bridier F, Bocher P, Savoie J. δ Phase precipitation in Inconel 718 and associated mechanical properties. Mater Sci Eng A. 2017;679:48–55. https://doi.org/10.1016/j.msea.2016.09.114 .
Basantia S, Singh V, Bhattacharya A, Khutia N, Das D. Prediction of tensile behaviour of ferrite-martensite dual phase steel using real microstructure-based RVE simulations. Mater Today Proc. 2018;5:18275–80. https://doi.org/10.1016/j.matpr.2018.06.165 .
Phetlam P, Uthaisangsuk V. Microstructure based flow stress modeling for quenched and tempered low alloy steel. Mater Des. 2015;82:189–99. https://doi.org/10.1016/j.matdes.2015.05.068 .
Sankanit P, Uthaisangsuk V, Pandee P. Tensile properties of hypoeutectic Al-Ni alloys: experiments and FE simulations. J Alloys Compd. 2021;889:161664. https://doi.org/10.1016/j.jallcom.2021.161664 .
Islam ST, Das P, Das S. Deformation behaviour of Rheocast A356 Al alloy at microlevel considering approximated RVEs. Met Mater Int. 2015;21:311–23. https://doi.org/10.1007/s12540-015-4234-z .
Taheri Andani M, Karamooz-Ravari MR, Mirzaeifar R, Ni J. Micromechanics modeling of metallic alloys 3D printed by selective laser melting. Mater Des. 2018;137:204–13. https://doi.org/10.1016/j.matdes.2017.10.026 .
Mikula J, Ahluwalia R, Laskowski R, Wang K, Vastola G, Zhang Y-W. Modelling the influence of process parameters on precipitate formation in powder-bed fusion additive manufacturing of IN718. Mater Des. 2021;207:109851. https://doi.org/10.1016/j.matdes.2021.109851 .
Malekipour K, Mashayekhi M, Badrossamay M. Meso-scale damage mechanics modeling for high cycle fatigue behavior of additively manufactured components. Mech Mater. 2021;160:103951. https://doi.org/10.1016/j.mechmat.2021.103951 .
Romanova V, Balokhonov R, Zinovieva O, Emelianova E, Dymnich E, Pisarev M, Zinoviev A. Micromechanical simulations of additively manufactured aluminum alloys. Comput Struct. 2021;244:106412. https://doi.org/10.1016/j.compstruc.2020.106412 .
Tang M, Pistorius PC, Beuth JL. Prediction of lack-of-fusion porosity for powder bed fusion. Addit Manuf. 2017;14:39–48. https://doi.org/10.1016/j.addma.2016.12.001 .
Scipioni Bertoli U, Wolfer AJ, Matthews MJ, Delplanque J-PR, Schoenung JM. On the limitations of volumetric energy density as a design parameter for selective laser melting. Mater Des. 2017;113:331–40. https://doi.org/10.1016/j.matdes.2016.10.037 .
Yadroitsev I, Gusarov A, Yadroitsava I, Smurov I. Single track formation in selective laser melting of metal powders. J Mater Process Technol. 2010;210:1624–31. https://doi.org/10.1016/j.jmatprotec.2010.05.010 .
Franco-Correa JC, Martínez-Franco E, Cruz-González CE, Salgado-López JM, Villada-Villalobos JA. Tailored time–temperature transformation diagram for IN718 alloy obtained via powder bed fusion additive manufacturing: phase behavior and precipitation dynamic. Materials. 2023;16:7280. https://doi.org/10.3390/ma16237280 .
Franco-Correa JC, Martínez-Franco E, Alvarado-Orozco JM, Cáceres-Díaz LA, Espinosa-Arbelaez DG, Villada JA. Effect of conventional heat treatments on the microstructure and microhardness of IN718 obtained by wrought and additive manufacturing. J Mater Eng Perform. 2021;30:7035–45. https://doi.org/10.1007/s11665-021-06138-9 .
Bayat M, Mohanty S, Hattel JH. Multiphysics modelling of lack-of-fusion voids formation and evolution in IN718 made by multi-track/multi-layer L-PBF. Int J Heat Mass Transf. 2019;139:95–114. https://doi.org/10.1016/j.ijheatmasstransfer.2019.05.003 .
Tillmann W, Schaak C, Nellesen J, Schaper M, Aydinöz ME, Hoyer KP. Hot isostatic pressing of IN718 components manufactured by selective laser melting. Addit Manuf. 2017;13:93–102. https://doi.org/10.1016/j.addma.2016.11.006 .
Kumar P, Farah J, Akram J, Teng C, Ginn J, Misra M. Influence of laser processing parameters on porosity in Inconel 718 during additive manufacturing. Int J Adv Manuf Technol. 2019;103:1497–507. https://doi.org/10.1007/s00170-019-03655-9 .
Li J, Zhao Z, Bai P, Qu H, Liu B, Li L, Wu L, Guan R, Liu H, Guo Z. Microstructural evolution and mechanical properties of IN718 alloy fabricated by selective laser melting following different heat treatments. J Alloys Compd. 2019;772:861–70. https://doi.org/10.1016/j.jallcom.2018.09.200 .
Ma M, Wang Z, Zeng X. Effect of energy input on microstructural evolution of direct laser fabricated IN718 alloy. Mater Charact. 2015;106:420–7. https://doi.org/10.1016/j.matchar.2015.06.027 .
Parimi LL, Clark RGAD, Attallah MM. Microstructural and texture development in direct laser fabricated IN718. Mater Charact. 2014;89:102–11. https://doi.org/10.1016/j.matchar.2013.12.012 .
Trosch T, Strößner J, Völkl R, Glatzel U. Microstructure and mechanical properties of selective laser melted Inconel 718 compared to forging and casting. Mater Lett. 2016;164:428–31. https://doi.org/10.1016/j.matlet.2015.10.136 .
Jiang R, Mostafaei A, Pauza J, Kantzos C, Rollett AD. Varied heat treatments and properties of laser powder bed printed Inconel 718. Mater Sci Eng A. 2019;755:170–80. https://doi.org/10.1016/j.msea.2019.03.103 .
Zhang F, Levine LE, Allen AJ, Stoudt MR, Lindwall G, Lass EA, Williams ME, Idell Y, Campbell CE. Effect of heat treatment on the microstructural evolution of a nickel-based superalloy additive-manufactured by laser powder bed fusion. Acta Mater. 2018;152:200–14. https://doi.org/10.1016/j.actamat.2018.03.017 .
Le W, Chen Z, Yan K, Naseem S, Zhao Y, Zhang H, Zhang Z. Early evolution of δ phase and coarse γ″ phase in Inconel 718 alloy with high temperature ageing. Mater Charact. 2021;180:111403. https://doi.org/10.1016/j.matchar.2021.111403 .
Shi X, Duan S-C, Yang W-S, Guo H-J, Guo J. Effect of cooling rate on microsegregation during solidification of superalloy INCONEL 718 under slow-cooled conditions. Metall Mater Trans B. 2018;49:1883–97. https://doi.org/10.1007/s11663-018-1169-z .
Kantzos C, Pauza J, Cunningham R, Narra SP, Beuth J, Rollett A. An investigation of process parameter modifications on additively manufactured Inconel 718 parts. J Mater Eng Perform. 2019;28:620–6. https://doi.org/10.1007/s11665-018-3612-3 .
Zhang D, Niu W, Cao X, Liu Z. Effect of standard heat treatment on the microstructure and mechanical properties of selective laser melting manufactured Inconel 718 superalloy. Mater Sci Eng A. 2015;644:32–40. https://doi.org/10.1016/j.msea.2015.06.021 .
Ormsuptave N, Uthaisangsuk V. Modeling of bake-hardening effect for fine grain bainite-aided dual phase steel. Mater Des. 2017;118:314–29. https://doi.org/10.1016/j.matdes.2017.01.040 .
El-Awady JA. Unravelling the physics of size-dependent dislocation-mediated plasticity. Nat Commun. 2015;6:5926. https://doi.org/10.1038/ncomms6926 .
Kumnorkaew T, Lian J, Uthaisangsuk V, Bleck W. Kinetic model of isothermal bainitic transformation of low carbon steels under ausforming conditions. Alloys. 2022;1:93–115. https://doi.org/10.3390/alloys1010007 .
Stoller RE, Zinkle SJ. On the relationship between uniaxial yield strength and resolved shear stress in polycrystalline materials. J Nucl Mater. 2000;283–287:349–52. https://doi.org/10.1016/S0022-3115(00)00378-0 .
Johansson J, Persson C, Testa G, Ruggiero A, Bonora N, Hörnqvist Colliander M. Effect of microstructure on dynamic shear localization in alloy 718. Mech Mater. 2017;109:88–100. https://doi.org/10.1016/j.mechmat.2017.03.020 .
Fisk M, Ion JC, Lindgren LE. Flow stress model for IN718 accounting for evolution of strengthening precipitates during thermal treatment. Comput Mater Sci. 2014;82:531–9. https://doi.org/10.1016/j.commatsci.2013.10.007 .
Wang J, Guo WG, Gao X, Su J. The third-type of strain aging and the constitutive modeling of a Q235B steel over a wide range of temperatures and strain rates. Int J Plast. 2015;65:85–107. https://doi.org/10.1016/j.ijplas.2014.08.017 .
Nemat-Nasser S, Li Y. Flow stress of F.C.C. polycrystals with application to OFHC Cu. Acta Mater. 1998;46:565–77. https://doi.org/10.1016/S1359-6454(97)00230-9 .
Holfelder P, Brenner F, Rund M, Witte A, Junghans S, Seyfert C, Richter M, Dell H, Koukolikova M, Gese H, Džugan J. Finite element simulation of plasticity and fracture for Inconel 718 deposited by laser powder bed fusion—chances, use and challenges. Addit Manuf. 2022;56:102888. https://doi.org/10.1016/j.addma.2022.102888 .
Huang L, Cao Y, Zhang J, Gao X, Li G, Wang Y. Effect of heat treatment on the microstructure evolution and mechanical behaviour of a selective laser melted Inconel 718 alloy. J Alloys Compd. 2021;865:158613. https://doi.org/10.1016/j.jallcom.2021.158613 .
Azadian S, Wei LY, Warren R. Delta phase precipitation in Inconel 718. Mater Charact. 2004;53:7–16. https://doi.org/10.1016/j.matchar.2004.07.004 .
Valle LCM, Araújo LS, Gabriel SB, Dille J, de Almeida LH. The effect of δ phase on the mechanical properties of an Inconel 718 superalloy. J Mater Eng Perform. 2013;22:1512–8. https://doi.org/10.1007/s11665-012-0433-7 .
Download references
Acknowledgements
The authors would like to acknowledge the financial supports of King Mongkut’s University of Technology Thonburi through the “Petchra Pra Jom Klao Scholarship” (Grant No. 7/2563), Thai Advanced Institute of Science and Technology (TAIST-Tokyo tech program), Thailand Science Research and Innovation (TSRI), National Science, Research and Innovation Fund (NSRF) through “Basic Research Fund: Fiscal year 2024”.
Author information
Authors and affiliations.
Center for Lightweight Materials, Design and Manufacturing, Department of Mechanical Engineering, Faculty of Engineering, King Mongkut’s University of Technology Thonburi, 126 Pracha Uthit Road, Bang Mod, Thung Khru, Bangkok, 10140, Thailand
P. Kumnaknoppakun & V. Uthaisangsuk
You can also search for this author in PubMed Google Scholar
Corresponding author
Correspondence to V. Uthaisangsuk .
Ethics declarations
Conflict of interest.
The authors have no conflicts of interest to declare that are relevant to the content of this article.
Ethical statement
The manuscript has been prepared by the contribution of all authors, it is the original authors work, it has not been published before, it has been solely submitted to this journal, and if accepted, it will not be submitted to any other journal in any language.
Additional information
Publisher's note.
Springer Nature remains neutral with regard to jurisdictional claims in published maps and institutional affiliations.
Rights and permissions
Springer Nature or its licensor (e.g. a society or other partner) holds exclusive rights to this article under a publishing agreement with the author(s) or other rightsholder(s); author self-archiving of the accepted manuscript version of this article is solely governed by the terms of such publishing agreement and applicable law.
Reprints and permissions
About this article
Kumnaknoppakun, P., Uthaisangsuk, V. Microstructure-based modelling of tensile properties of heat treated Inconel 718 alloy produced by laser powder bed fusion. Arch. Civ. Mech. Eng. 24 , 227 (2024). https://doi.org/10.1007/s43452-024-01034-0
Download citation
Received : 28 April 2024
Revised : 20 July 2024
Accepted : 17 August 2024
Published : 05 September 2024
DOI : https://doi.org/10.1007/s43452-024-01034-0
Share this article
Anyone you share the following link with will be able to read this content:
Sorry, a shareable link is not currently available for this article.
Provided by the Springer Nature SharedIt content-sharing initiative
- Inconel 718
- Microstructure
- Flow stress curve
- Find a journal
- Publish with us
- Track your research

IMAGES
VIDEO
COMMENTS
Incidentally, humans are sensitive to heat flow rather than to temperature. Since heat is a form of energy, its SI unit is the joule (J). Another common unit of energy often used for heat is the calorie (cal), defined as the energy needed to change the temperature of 1.00 g of water by 1.00 ° C 1.00 ° C —specifically, between 14.5 ° C 14.5 ...
The Heat Flow Experiment was a United States NASA lunar science experiment that aimed to measure the rate of heat loss from the surface of the Moon.Four experiments were carried out on board Apollo missions. Two experiments were successfully deployed as part of Apollo 15 and Apollo 17.The instrument on Apollo 16 was deployed but the cable from it to the ALSEP central station was broken and the ...
where m is the mass of the substance and ΔT is the change in its temperature, in units of Celsius or Kelvin.The symbol c stands for specific heat, and depends on the material and phase.The specific heat is the amount of heat necessary to change the temperature of 1.00 kg of mass by 1.00 ºC. The specific heat c is a property of the substance; its SI unit is J/(kg ⋅ ⋅ K) or J/(kg ⋅ ⋅ ...
Finally, we have explored a thought experiment in which a metal can containing hot water is placed within a Styrofoam cup containing cold water. Heat is transferred from the hot water to the cold water until both samples have the same temperature. ... Conductive heat flow involves the transfer of heat from one location to another in the absence ...
1.6 Mechanisms of Heat Transfer - University Physics ...
Thermal resistance is a heat property and the measurement by which an object or material resists to heat flow (heat per time unit or thermal resistance) to temperature difference. Radiance, ... which he describes in great detail in the Philosophical Transactions article "New Experiments upon Heat" from 1786. [52] ...
Now repeat the experiment for different materials (cold water (30 °C), hot water (50 °C) aluminum foil, soil, corn syrup, glue) Convection: Fill ¾th of the beaker with water at room temperature. Fill the conical flask with water and add ice. Mix well to get cold water and then add a food coloring agent.
Place the ice bath on top of the sandwich. Switch the hotplate controller on and set the Variac to approximately 40% power. The exact value is not important, but if the power is set much higher some of the materials may get too hot. It will take up to 30 minutes for the heat flow to achieve a steady state.
When Heat Flows, Is Something Material Actually Flowing? By the late 1700's, the experiments of Fahrenheit, Black and others had established a systematic, quantitative way of measuring temperatures, heat flows and heat capacities—but this didn't really throw any new light on just what. was flowing. This was a time when the study of ...
Heat Transfer - Conduction, Convection, Radiation
The heat flow is more rapid at first as depicted by the steeper slopes of the lines. ... Joule measured both the amount of mechanical work done and the amount of heat gained by the water. Similar experiments demonstrating that heat could be generated by an electric current dealt a further blow to the thought that heat was a fluid that was ...
Conducting Heat Science Experiment Instructions. Step 1 - Begin by positioning 3 spoons in a small glass bowl. Step 2 - Place a small pat of butter at the top of each spoon. Step 3 - Put a bead in each pat of butter. Step 4 - Carefully pour hot boiling water into the bowl until it is almost completely full. Be careful not to allow the ...
Experiment reverses the direction of heat flow. Heat flows from hot to cold objects. When a hot and a cold body are in thermal contact, they exchange heat energy until they reach thermal ...
043:15:39 - This is Apollo Control at 43 hours, 16 minutes. Apollo 17 is 145,178 nautical miles [268,870 km] from Earth, traveling at a speed of 3,543 feet per second [1,080 m/s]. The crew is now in the midst of the heat flow and convection demonstration. This is the demonstration that's designed to provide more exact data on the behavior of ...
It was recently shown theoretically that the time-dependent heat conduction equation is form invariant under curvilinear coordinate transformations. Thus, in analogy to transformation optics, fictitious transformed space can be mapped onto (meta)materials with spatially inhomogeneous and anisotropic heat-conductivity tensors in the laboratory space. On this basis, we design, fabricate, and ...
The heat flow experiment data from 1975 to 1977 had not been archived with the National Space Science Data Center . Recently, major portions of the 1975 - 1977 heat flow data h ave been restored from the original archival datatapes for the ALSEP experi-
Experiments conducted aboard Apollo 17 by astronaut Ronald E. Evans showed that in uncovered liquids convection driven by surface tension can occur at lower temperature gradients in low gravity (about 10-8 g) than in 1g.In completely confined fluids (no liquid-gas interface) vibrations caused by spacecraft and astronaut movements increased the heat transfer considerably over the pure ...
For example, Owen et al. [26] visualized results from towing tank experiments to study flow past a sinuous bluff body with a circular cross-section at Re ... To the best of our knowledge, this is the first systematic numerical and experimental investigation of the flow field and heat transfer around a zigzag eddy promoter in a confined domain.
The uneven distribution of flow phases in evaporator channels can drop the heat exchanger efficiency up to 30%. Due to its dependence on the interaction of seve. ... this is an inefficient and expensive way of doing experiments, and the results lack in understanding how the combination of variables affects the flow distribution. ...
Within fluid mechanics, the flow of hybrid nanofluids over a stretching surface has been extensively researched due to their influence on the flow and heat transfer properties. Expanding on this ...
It was found that predicted flow stress curves fairly agreed with those from the experiments. Increasing amount and size of the δ phase in the printed Inconel samples by longer DPT holding time led to decreased strength due to the depletion of strengthening γ′′ phase, but enhanced strain hardening because of the pinning effect on ...