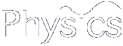
- Collections
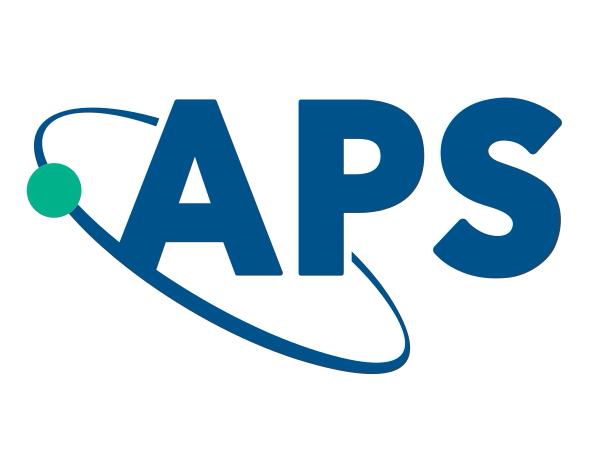
- APS Journals

Revamp for High-Pressure-Superconductivity Measurements
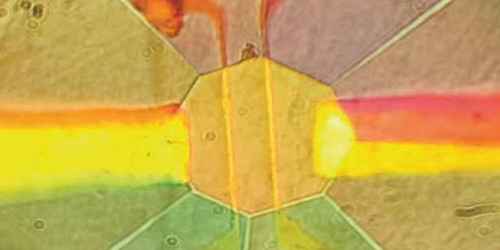
At pressures above 90 gigapascals (GPa), sulfur switches from behaving like a nonmetal to behaving like a metal. Lower the temperature and the highly pressurized metal then starts superconducting. Reaching such prodigious pressures requires placing the sulfur in a diamond-anvil cell, a device that previously precluded the making of all but the simplest measurements of the system. Now, however, Feng Du of the Max Planck Institute of Chemistry, Germany, and his collaborators have instrumented a sulfur sample held at a pressure of up to 160 GPa [ 1 ]. Their achievement could allow researchers to probe the high-pressure properties of the growing number of materials that have recently been found to superconduct in this pressure regime.
At a pressure of 160 GPa and a temperature of 17 K, electrons in sulfur pair up, forming a collective state that can flow without resistance. The further the temperature drops below 17 K, the more energy is required to cleave the paired electrons, making the superconducting state more robust.
Theory connects the size of the cleaving energy, which is known as the superconducting gap, to the magnitude of the current that tunnels from a normal conductor to a superconductor when the two materials sit on either side of an insulating barrier. Du and his collaborators fashioned such a “tunnel” junction from sulfur (superconductor), tantalum (metal), and tantalum pentoxide (insulator). The choice of insulator was crucial. Tantalum pentoxide’s high density and stability ensured that the layer’s thickness would not change under the high pressure.
Using this setup, Du and his collaborators measured sulfur’s superconducting gap, finding a value that matched theoretical predictions. The researchers say that they look forward to using their tunnel junction design with more exotic, less understood materials, such as hydrides and nickelates.
–Charles Day
Charles Day is a Senior Editor for Physics Magazine .
- F. Du et al. , “Tunneling spectroscopy at megabar pressures: Determination of the superconducting gap in sulfur,” Phys. Rev. Lett. 133 , 036002 (2024) .
Tunneling Spectroscopy at Megabar Pressures: Determination of the Superconducting Gap in Sulfur
F. Du, F. F. Balakirev, V. S. Minkov, G. A. Smith, B. Maiorov, P. P. Kong, A. P. Drozdov, and M. I. Eremets
Phys. Rev. Lett. 133 , 036002 (2024)
Published July 17, 2024
Subject Areas
Related articles.
Golden Ratio in Quasicrystal Vibrations
Experiments show that a property of the vibrations in a quasicrystal is linked to the number known as the golden ratio. Read More »
Atomic Friction Defies Expectations
An experiment reveals that frictional forces can have a surprisingly complex velocity dependence at the nanoscale. Read More »
Analyzing Friction in Layered Materials
Experiments reveal the factors that determine the friction between the single-atom-thick layers in van der Waals materials, which may have uses in lubrication technology. Read More »
Sign up to receive weekly email alerts from Physics Magazine .
Help | Advanced Search
Condensed Matter > Superconductivity
Title: advanced approach of superconducting gap function extraction from tunneling experiments.
Abstract: An advanced theoretical framework is introduced and examined. Its main idea is to extract properties of the superconducting pairing gap function $\Delta(\omega)$ in the conventional, nearly localized superconductors. To test the approach, we present an experimentally relevant benchmark model with defined normal and superconducting sectors. The developed reverse engineering framework consists of two logic steps. First, dismantle the superconducting density of states into the effects coming from the superconducting pairing and effects inherited from the normal state. Second, extract and reconstruct properties of $\Delta(\omega)$ and compare it to the superconducting sector of the defined benchmark model. Applying this approach, we can: (i) simulate extraction from the actual experimental low-temperature tunneling data and comment on their required properties, and (ii) maintain absolute control above the reconstructed Cooper-pair-influencing properties during ameliorating the individual steps of the method.
Comments: | 15 pages (10 pages main text + 5 pages appendixes), 17 figures |
Subjects: | Superconductivity (cond-mat.supr-con) |
Cite as: | [cond-mat.supr-con] |
(or [cond-mat.supr-con] for this version) | |
Focus to learn more arXiv-issued DOI via DataCite | |
: | Focus to learn more DOI(s) linking to related resources |
Submission history
Access paper:.
- Other Formats
References & Citations
- Google Scholar
- Semantic Scholar
BibTeX formatted citation

Bibliographic and Citation Tools
Code, data and media associated with this article, recommenders and search tools.
- Institution
arXivLabs: experimental projects with community collaborators
arXivLabs is a framework that allows collaborators to develop and share new arXiv features directly on our website.
Both individuals and organizations that work with arXivLabs have embraced and accepted our values of openness, community, excellence, and user data privacy. arXiv is committed to these values and only works with partners that adhere to them.
Have an idea for a project that will add value for arXiv's community? Learn more about arXivLabs .

An official website of the United States government
The .gov means it’s official. Federal government websites often end in .gov or .mil. Before sharing sensitive information, make sure you’re on a federal government site.
The site is secure. The https:// ensures that you are connecting to the official website and that any information you provide is encrypted and transmitted securely.
- Publications
- Account settings
The PMC website is updating on October 15, 2024. Learn More or Try it out now .
- Advanced Search
- Journal List
- PMC11133361
ARPES detection of superconducting gap sign in unconventional superconductors
1 Beijing National Laboratory for Condensed Matter Physics, Institute of Physics, Chinese Academy of Sciences, Beijing, 100190 China
2 Department of Physics, Pohang University of Science and Technology (POSTECH), Pohang, 37673 Korea
3 Beijing Academy of Quantum Information Sciences, Beijing, 100193 China
Hongtao Yan
Xiangyu luo.
4 School of Physical Sciences, University of Chinese Academy of Sciences, Beijing, 100049 China
Yongqing Cai
Chaohui yin.
5 Condensed Matter Physics and Materials Science Department, Brookhaven National Laboratory, Upton, NY 11973 USA
Fengfeng Zhang
6 Technical Institute of Physics and Chemistry, Chinese Academy of Sciences, Beijing, 100190 China
Shenjin Zhang
Qinjun peng.
7 Songshan Lake Materials Laboratory, Dongguan, Guangdong 523808 China
Guodong Liu
Han-yong choi.
8 Department of Physics and Institute for Basic Science Research, SungKyunKwan University, Suwon, 440-746 Korea
Associated Data
All data are processed by Igor Pro 8.0.2 software. All data needed to evaluate the conclusions in the paper are available within the article and its Supplementary Information files. All data generated during the current study are available from the corresponding author upon request.
The codes used for the calculations in this study are available from the corresponding authors upon request.
The superconducting gap symmetry is crucial in understanding the underlying superconductivity mechanism. Angle-resolved photoemission spectroscopy (ARPES) has played a key role in determining the gap symmetry in unconventional superconductors. However, it has been considered so far that ARPES can only measure the magnitude of the superconducting gap but not its phase; the phase has to be detected by other phase-sensitive techniques. Here we propose a method to directly detect the superconducting gap sign by ARPES. This method is successfully validated in a cuprate superconductor Bi 2 Sr 2 CaCu 2 O 8+ δ with a well-known d -wave gap symmetry. When two bands have a strong interband interaction, the resulted electronic structures in the superconducting state are sensitive to the relative gap sign between the two bands. Our present work provides an approach to detect the gap sign and can be applied to various superconductors, particularly those with multiple orbitals like the iron-based superconductors.
According to conventional wisdom, angle-resolved photoemission spectroscopy (ARPES) can only measure the magnitude of the superconducting gap but not its phase. Here, the authors propose a new method to directly detect the superconducting gap phase using ARPES and validate this technique on a cuprate superconductor.
Introduction
The superconducting gap is the most basic and important physical quantity of superconductors which is characterized by the magnitude and sign. Its determination is essential for understanding the mechanism of superconductivity. While the conventional superconductors exhibit an s -wave gap symmetry that has the same sign along the entire Fermi surface, in unconventional superconductors, the superconducting gap may have different signs on different parts of the Fermi surface 1 . High temperature cuprate superconductors have been extensively studied for more than thirty years due to its unusually high critical temperature ( T c ), anomalous normal state, and challenging mechanism of high temperature superconductivity 2 – 6 . One of the most significant achievements is the establishment of the d -wave pairing symmetry that pinpoints the unconventional superconductivity mechanism in the cuprate superconductors 2 . For the magnitude of the superconducting gap, angle-resolved photoemission spectroscopy (ARPES) played an important role in directly determining the anisotropic gap size in the momentum space that is consistent with the d -wave symmetry 4 , 7 , 8 . However, the ARPES measurements do not provide the sign information that is necessary in pinning down the pairing symmetry. For the phase information of the d -wave gap, it was obtained later on by the phase-sensitive experiments based on Josephson tunneling and flux quantization 2 , 9 , 10 that were specially designed for the cuprate superconductors which have relatively simple Fermi surface and d -wave superconducting gap (Fig. 1 a). These methods have not been successfully applied to the other superconductors like the iron-based superconductors which possess multiple Fermi surface sheets and possible unconventional pairing with gap sign changes (Fig. 1 b) 11 – 13 . So far, experimental extraction of the sign information in the gap function has proven to be significant but challenging in unconventional superconductors 1 . It has been attempted in the scanning tunneling microscopy (STM) measurements utilizing quasiparticle interference 14 – 18 . Although ARPES is a powerful tool to directly measure the superconducting gap magnitude, it has long been believed that it can not probe the sign of the superconducting gap and therefore there has been no ARPES report in the sign detection of the superconducting gap.

a Schematic Fermi surface of cuprate superconductors. The superconducting gap has a d -wave form which exhibits a sign change along the nodal directions. b Typical Fermi surface of the iron-based superconductors with multiple hole and electron pockets. c – i Proposed method to determine the relative sign of the superconducting gaps between two bands. c shows the initial two bare bands that are degenerate ( α i and β i ). d shows the simulated α and β bands in the normal state after putting the interband coupling ( V = 10 meV) with the Fermi momenta marked. e shows the simulated band structures in the superconducting state with a fixed V = 10 meV and increasing initial gap size Δ i . Here, the same magnitude and the same sign of the initial superconducting gaps are taken for the two bands. Superconducting gap (2Δ α and 2Δ β ) opens at the Fermi momenta k F α and k F β , yielding four branches of bands labeled as α U , β U , α L and β L . The Bogoliubov backbending band of α L crosses the β L band at a momentum k H as marked by red arrows. f Same as e but the opposite sign of the initial superconducting gaps are taken for the two bands. The Fermi momentum is no longer conserved from the normal state. When defined as the momentum that is close to the Fermi level, the Fermi momentum changes dramatically with the variation of Δ i . The superconducting gap becomes also significantly different from the general picture in e . Pronounced Bogoliubov hybridization gap (Δ h ) opens at k H in this case while it is basically zero in e . g Evolution of the Fermi momenta of the α and β bands with Δ i in the normal and superconducting states where the same and opposite signs of the two initial superconducting gaps are considered. h Evolution of the superconducting gap Δ α and Δ β with Δ i when the two initial gaps have the same sign and opposite sign. i Evolution of the hybridization gap Δ h with Δ i when the two initial gaps have the same sign and opposite sign.
In this work, we develop a method to detect superconducting gap sign by ARPES. It is motivated by our observation of an unusual Bogoliubov band hybridization in the cuprate superconductor Bi 2 Sr 2 CaCu 2 O 8+ δ (Bi2212) 19 . The gap sign manifests itself in the resulted electronic structures in the superconducting state including the Fermi momentum shift, the strong Bogoliubov band hybridization and the abnormal superconducting gap behaviors. The proposed method is well tested in the ARPES measurements of Bi2212 with a d -wave gap symmetry. The present work provides the approach to detect the superconducting gap sign which is significant for understanding the mechanism of unconventional superconductors.
Proposed method
To detect the relative sign of the superconducting gap between two bands, we propose a method based on the Bogoliubov band hybridization. For a system with two bands ( α and β ) which are close in momentum space (Fig. 1 c–f), its superconducting state can be described by a phenomenological Hamiltonian
where ε i α and ε i β represent the initial α and β bare bands, V is the coupling strength between the two bands, and Δ i α and Δ i β are the initial superconducting gap of the α and β bands 20 , 21 . Such a Hamiltonian can also describe the normal state when the initial superconducting gaps are taken as zeros.
Figure 1 c–i shows the simulated band structures of the two band system in the normal state and in the superconducting state (see Supplementary Note 1 for the details of the simulation). To be typical and for simplicity, we started with the two initial bare bands which are degenerate (Fig. 1 c). When there is an interband coupling ( V = 10 meV) between the two bands, the band structure in the normal state exhibits a band splitting (Fig. 1 d). In the superconducting state, the relative sign of the initial superconducting gap between the two bands dramatically affects the resulted band structures. When the initial superconducting gap of the two bands is taken as the same both in the magnitude and in its sign (Fig. 1 e), superconducting gap opens in the normal way at the two Fermi momenta and there is no noticeable hybridization between the Bogoliubov backbending band of α and the β band at the crossing point k H . In contrast, when the initial superconducting gap of the two bands takes the opposite sign, the resulted band structures (Fig. 1 f) become totally different from those in Fig. 1 e in terms of the unusual change of the Fermi momentum (Fig. 1 g), the superconducting gap (Fig. 1 h) and the opening of a hybridization gap at k H (Fig. 1 i). While the two Fermi momenta keep fixed in the superconducting state when the two initial superconducting gaps have the same sign, they can be dramatically shifted in the case of the opposite gap sign (Fig. 1 g). Depending on the relative magnitude of the initial superconducting gap (Δ i ) and the interband coupling ( V ), the two Fermi momenta in the normal state may even evolve into the same one in the superconducting state. In the case of the superconducting gap, although it keeps the same with the initial superconducting gap in the superconducting state when the two initial superconducting gaps have the same sign, it is completely altered when the two initial superconducting gaps take the opposite sign (Fig. 1 h). The superconducting gap may even become zero in the superconducting state when the initial superconducting gap (Δ i ) is relatively smaller than the interband coupling ( V ). Whereas the band hybridization gap is nearly zero when the two initial superconducting gaps have the same sign, it can become significant in the case of the opposite gap sign in the superconducting state (Fig. 1 i). These fundamental differences in the resulted band structures form the basis of our proposed method to probe the relative gap sign between the two bands through the ARPES measurements.
We just discussed one typical and extreme case where the initial two bare bands are degenerate. We also carried out simulations on the cases that the initial two bare bands are different, in particular, how the Bogoliubov band hybridization evolves with the separation of the two bare bands (Supplementary Fig. 1) . It is found that the dramatic difference of the electronic structures in the superconducting state between the same-gap-sign and the opposite-gap-sign cases still persists. Even if the initial two bare bands separate, when the initial two superconducting gaps have the opposite sign, the unusual behaviors like the Fermi momentum shift, the pronounced Bogoliubov band hybridization and the abnormal superconducting gap remain present. This indicates that our proposed method is more general which can be used for both cases that the initial two bare bands are degenerate and separate.
Manifestations of gap sign in Bogoliubov band hybridization
In order to test the above proposed method, it is necessary to find a superconductor that is unconventional and its superconducting gap symmetry is well established with a sign change. Such superconductors are rare and cuprate superconductors are essentially the only known case that can satisfy the stringent requirements. It is well known that the cuprate superconductors have a d -wave superconducting gap that changes sign on different parts of the Fermi surface (Fig. 1 a). Furthermore, to test the method in the cuprate superconductors, it is also necessary to find two bands that are close in momentum space and have the opposite gap sign.
In the process of studying the origin of the superstructure bands in Bi2212 19 , we came across an ideal case that can test our proposed method. In Bi2212, because of the presence of the incommensurate structural modulations, in addition to the main bonding and antibonding bands, superstructure bands are formed by shifting the main bands with the superstructure wavevector, ± Q , as shown in Fig. 2 a and Supplementary Fig. 2 19 , 22 , 23 . While the main Fermi surface and the superstructure Fermi surface are well separated in the first quadrant, they cross each other in the second quadrant (Fig. 2 a). Figure 2 b shows a constant energy contour near the Fermi level covering the band crossing area in the second quadrant. Here mainly two Fermi surface sheets are observed due to the photoemission matrix element effects: the main antibonding Fermi surface sheet(AB) and the superstructure antibonding Fermi surface sheet(AB_SS). It has been found that the main bands and the superstructure bands exhibit a selective band hybridization, i.e., the initial main antibonding band (AB, red line in Fig. 2 b) hybridizes with the initial superstructure bonding band (BB_SS, dashed blue line in Fig. 2 b) 19 , 24 , as shown in Fig. 2 b. The main AB Fermi surface is then broken into two branches (Branch1 and Branch2) at the crossing point MS induced by the hybridization. Therefore, we have found a rare but ideal case to test our method in Bi2212: (1), There are two bands, the main antibonding band (AB) and the superstructure bonding band (BB_SS), that are close-by in momentum space; (2), These two bands exhibit strong interband coupling; (3), The superconducting gap sign of the two bands is opposite; and (4), The superconducting gap magnitude of the two bands is similar.

a Schematic Fermi surface of Bi2212 that is composed of the main Fermi surface (thick lines, antibonding (AB) and bonding (BB)) and the superstructure Fermi surface (thin lines, AB_SS and BB_SS). The superstructure Fermi surface is obtained by shifting the main Fermi surface with a superstructure wave vector ± Q along Γ-Y. The superconducting gap sign is marked by blue (positive) and red (negative). b Constant energy contour at the binding energy of 15 meV measured at 15 K. The covered momentum space (dashed square in a ) lies in the second quadrant. The initial AB (Ini. AB) and BB_SS (Ini. BB_SS) cross at a point MS. The main AB is broken into two branches (Branch1 and Branch2) at MS due to its hybridization with the superstructure BB_SS. c Band structure in the normal state measured at 80 K along the momentum cut Cut1. It is a second derivative image with respect to the momentum. The momentum cut position is marked in b by the black line with a Fermi surface angle θ = 32, as defined in a . Two bands are observed corresponding to the Branch1 (BR1, pink line) and Branch2 (BR2, purple line) Fermi surface in b . The origin of the momentum axis is defined at the zone corner. We adopt this definition throughout the paper. d Same as c but measured in the superconducting state at 15 K. The image is acquired by dividing the Fermi distribution function. e The energy distribution curves (EDCs) of d . f – i Simulated band structures with an interband coupling. f shows two initial bare bands without band coupling. g shows two bands in the normal state after putting V = 12 meV. h shows the band structure in the superconducting state. The initial superconducting gaps of the two bands are assumed to be Δ i α = 11.6 meV and Δ i β = −5.8 meV with the opposite sign. i Same as h but the initial superconducting gaps are assumed to have the same sign: Δ i α = 11.6 meV and Δ i β = 5.8 meV. j EDCs of h .
Figure 2 shows the band structures of Bi2212 measured in the normal state (Fig. 2 c) and the superconducting state (Fig. 2 d) along a typical momentum cut near the crossing region of the AB and BB_SS Fermi surface. In the normal state, two bands are mainly observed, labeled as BR1 and BR2 in Fig. 2 c, that correspond to Branch1 and Branch2 Fermi surface in Fig. 2 b. In the superconducting state, the observed band structure (Fig. 2 d) is strikingly different from generally expected picture that superconducting gaps open at the two Fermi momenta. It is unusual in several aspects. First, there is an obvious Fermi momentum shift in the superconducting state. The Fermi momentum separation between the two bands shrinks from 0.023 π / a in the normal state to 0.018 π / a in the superconducting state. Second, the gap opening at the two Fermi momenta is quite unusual. The particle-hole symmetry is not conserved at the Fermi momentum as seen from the BR2 band in Fig. 2 d and the photoemission spectrum (energy distribution curve, EDC) at the BR2 Fermi momentum (purple line in Fig. 2 e). The gap center is obviously away from the chemical potential. This is not caused by the improper Fermi function division 25 because the energy resolution we used is rather high (~1 meV). Third, below the Fermi level, the BR2 band breaks into two parts with a strong spectral weight suppression around the binding energy of 14 meV, as marked by red arrows in Fig. 2 d. Such a band breaking and the dramatic spectral weight suppression of the BR2 band can also be seen from the corresponding EDCs in the superconducting state (Fig. 2 e).
The unusual behaviors observed in the superconducting state (Fig. 2 d) can be understood in terms of the two band model we proposed in Equation (1) by taking proper bare bands, initial superconducting gaps and the coupling strength between the two bands. Figure 2 f–i shows the simulated band structure in the normal state and superconducting state. In the simulation process, the relative sign of the initial superconducting gap between the two bands plays a decisive role in dictating the band structures in the superconducting state. Figure 2 h shows the simulated band structure in the superconducting state by considering the opposite sign of the superconducting gaps on the two bands. The corresponding EDCs are shown in Fig. 2 j. The simulated results (Fig. 2 h, j) are highly consistent, even on the quantitative level, with the measured band structure (Fig. 2 d) and EDCs (Fig. 2 e). All the unusual behaviors observed in the superconducting state are well captured by the simulations. In contrast, if the same sign of the superconducting gap is taken for the two bands, the simulated band structure (Fig. 2 i) deviates far from the measured result (Fig. 2 d). The observation of the unusual band structures in the superconducting state and their quantitative understanding based on the two band model indicate unambiguously that the superconducting gaps of the two bands have the opposite sign. It demonstrates the feasibility of our proposed method in detecting the relative sign of the superconducting gaps on two bands. This is the first time that the superconducting gap sign is detected from ARPES measurements.
The relative gap sign also manifests itself in the Fermi surface topology, momentum dependence of the band structure, and the associated momentum dependence of the Bogoliubov band hybridization. Figure 3 a shows the detailed Fermi surface mapping in the superconducting state around the crossing point MS of the initial main AB and the superstructure BB_SS Fermi surface. The hybridization between the AB and BB_SS bands results in breaking the main AB Fermi surface into two branches (Branch1 and Branch2) with the spectral weight enhanced near the crossing point. Figure 3 b shows band structures measured along different cuts in the covered momentum space in Fig. 3 a, c (more complete momentum-dependent band structures are shown in Supplementary Fig. 3 ). Within a narrow momentum space, the observed band structures exhibit a dramatic and systematic momentum dependence. Moreover, like the band structure for θ = 32 that is analyzed in detail in Fig. 2 , these observed bands are also unusual in the gap opening and Bogoliubov band hybridization. The BR1 band shows a strong Bogoliubov hybridization with the BR2 band near the crossing point MS (Cut1 and Cut2 in Fig. 3 b) and the hybridization gets weaker with the momentum cuts moving away from MS (Cut3 to Cut5 in Fig. 3 b). The quantitative evolution of the Bogoliubov hybridization gap with momentum is extracted in Supplementary Fig. 4 .

a Fermi surface mapping of Bi2212 measured at 15 K. The covered momentum space is in the second quadrant as shown by the dashed black frame in c . The AB Fermi surface is mainly observed but is broken into two branches, Branch1 (pink line) and Branch2 (purple line), due to hybridization with the superstructure BB_SS Fermi surface. b Band structures measured along different momentum cuts around the crossing area of the initial Fermi surface AB and BB_SS. The images are obtained by dividing the Fermi distribution function to show part of the band structure above the Fermi level. The location of the momentum cuts is shown by the solid black lines in c and also be defined by the Fermi surface angle, θ . Two bands are mainly observed as marked by pink arrows for the Branch1 (BR1) and purple arrows for the Branch2 (BR2). c Schematic Fermi surface in the second quadrant with the related initial main AB Fermi surface and the superstructure BB_SS Fermi surface plotted. The gap sign on the Fermi surface is marked by the red color for the positive and the blue color for the negative by considering d -wave pairing symmetry. d Simulated Fermi surface of Bi2212 by considering the opposite gap sign on the AB and BB_SS Fermi surface in the Fermi surface crossing area. e The corresponding simulated band structures along different momentum cuts. f , g Same as d , e but by considering the same gap sign on the AB and BB_SS Fermi surface. h Momentum dependence of the Bogoliubov hybridization gap Δ h . The definition of Δ h is schematically shown in the upper insets. The measured values (see Supplementary Fig. 4 ) are plotted as black circles. The red (blue) line shows the simulated Δ h by considering the opposite (same) gap sign of the main band AB and the superstructure band BB_SS. Error bars are determined based on the fitting error of the EDC peak position.
The measured Fermi surface mapping (Fig. 3 a) and the unusual momentum-dependent band structure evolution (Fig. 3 b) can be understood by the two band model (Equation (1)) only when the relative sign of the superconducting gaps on the two bands are taken opposite. We note that, in the particular case of Bi2212, the initial two bare bands and the initial two superconducting gap sizes are known beforehand which can be determined from the measurements on the two main bands (AB and BB) in the first quadrant (see Supplementary Fig. 5 , Supplementary Fig. 6 and the tight binding fitting in Supplementary Note 2 ). In principle, the coupling strength V between the main AB band and the superstructure BB_SS band can also be determined from the band structure measurements in the normal state 19 . Here we take it as a constant in the small covered momentum space of the Fermi surface crossing region. Under the condition that all the parameters in Equation (1) are known, we globally simulated the Fermi surface and momentum-dependent band structures by considering the opposite gap sign (Fig. 3 d, e) and the same gap sign (Fig. 3 f, g) for the two bands. When the two gaps take the opposite sign, the simulated Fermi surface mapping (Fig. 3 d) well reproduces the measured Fermi surface in Fig. 3 a. The momentum-dependent band structures and the associated Bogoliubov band hybridization (Fig. 3 b) are well captured in the simulated results (Fig. 3 e). In particular, the measured momentum-dependent Bogoliubov hybridization gap (black circles in Fig. 3 h) shows a quantitative agreement with the simulations (red line in Fig. 3 h). In contrast, if the same gap sign is taken for the two bands, the simulated Fermi surface (Fig. 3 f), the overall momentum-dependent band structures (Fig. 3 g) and the Bogoliubov hybridization gap (blue line in Fig. 3 h) deviate significantly from the measured results. These results lend further decisive evidence that the relative sign of the superconducting gap on the two bands is opposite in the covered momentum space.
Manifestations of gap sign in the abnormal superconducting gap
As shown from the simulations of the two band model in Fig. 1 and Supplementary Fig. 1 , one of the main signatures of the relative gap sign is the unusual superconducting gap behaviors. Such an anomaly of the superconducting gap is observed in Bi2212 in the crossing area of two bands with opposite gap sign (Fig. 4 a). Figure 4 b highlights the measured band structure near the Fermi level along the momentum cut of θ =32 in the superconducting state. The corresponding original EDCs and the EDCs after dividing the Fermi distribution function at the two Fermi momenta (BR1_k F and BR2_k F ) are shown in Fig. 4 c–f, respectively. The superconducting gaps of the two bands are unusual in several aspects. First, the BR1 band crosses the Fermi level with a gap size that is nearly zero. Second, the particle-hole symmetry for the BR2 band is apparently broken where the spectral weight at the Fermi momentum is not symmetrical with respect to the Fermi level. Third, compared with the gap size at the equivalent momentum position in the first quadrant, the gap size of the BR1 and BR2 bands after the hybridization in the second quadrant is much reduced from the initial 5.8 meV and 11.6 meV to 0 meV and 5 meV, respectively. As shown in Fig. 2 h, these unusual gap behaviors can be well reproduced when the relative gap sign of the two bands is opposite. We note that the particle-hole symmetry breaking manifests itself in the dramatic difference of the spectral weight distribution with respect to the chemical potential but the energy positions remain symmetric with respect to the chemical potential. Since the superconducting gap size is determined from the EDC peak position, the broken particle-hole symmetry does not affect our determination of the superconducting gap even near the nodal region.

a Fermi surface mapping of Bi2212 in the second quadrant measured at 15 K. It is the MDC second derivative image. b Band structure measured at 15 K along the momentum cut (Cut1 in a ) with the Fermi surface angle θ = 32. The image is obtained by dividing the Fermi distribution function. c , d Original EDCs at the Fermi momenta of the BR1 and BR2 bands, respectively, obtained from b . e , f Corresponding EDCs of c , d after dividing the Fermi distribution function. The superconducting gap size is nearly zero for the BR1 band and 5 meV for the BR2 band. g , h EDCs from the Branch1 and Branch2 parts of Fermi surface, respectively, obtained by dividing the Fermi distribution function. The location of the Fermi momentum points is shown by the pink open circles for the Branch1 and purple open circles for the Branch2 in a which is also defined by the angle θ . i Momentum dependence of the superconducting gap on the two branches of Fermi surface. The superconducting gaps on the Branch1 (pink open circles) and the Branch2 (purple open circles) are obtained from the EDCs in g , h . Error bars are determined based on the fitting error of the EDC peak position. The dashed thick black line represents the usual d -wave superconducting gap ( Δ = 29 * ∣ cos k x − cos k y ∣ / 2 ) on the AB Fermi surface as obtained in the first quadrant (Supplementary Fig. 6 d). The dashed thin black line represents the usual superconducting gap on the BB_SS Fermi surface. Since the superstructure Fermi surface is shifted by a wavevector Q along the Γ-Y direction relative to the main Fermi surface, the nodal point in the second quadrant is shifted from the initial θ = 45 for the main Fermi surface to θ = 26.5 for the superstructure Fermi surface, as shown by the dashed thin black line in the top insets. The solid pink line and solid purple line represent the simulated superconducting gaps on the Branch1 and Branch2 Fermi surface, respectively, by considering the Bogoliubov band hybridization between the two bands.
Figure 4g, h shows EDCs measured along the BR1 and BR2 Fermi surface in the superconducting state. The momentum dependent superconducting gap along the two branches of Fermi surface is plotted in Fig. 4 i. Near the crossing area, the measured superconducting gap strongly deviates from the standard d -wave form with the gap size much reduced and even new accidental nodes can be produced. The unusual momentum dependence of the superconducting gap can be well understood by considering the two bands hybridization and the opposite gap sign on the two bands. As shown in Fig. 4 i, the superconducting gap obtained from the same global band structure simulations (Fig. 3 e) is quantitatively consistent with the measured results. This further demonstrates that the relative sign of the superconducting gap on the two bands is opposite in the covered momentum space.
Our present results show that the hybridization between two bands with opposite superconducting gap sign can induce unusual behaviors. First, the Fermi momenta can be shifted in the superconducting state relative to those in the normal state (Fig. 2 c, d). Second, the density of states at the Fermi momenta may become unsymmetrical with respect to the Fermi level which indicates the particle-hole symmetry is no longer conserved in the superconducting state (Fig. 2 e). Third, it can produce gap nodes even though the initial gap of the two bands is non-zero (Fig. 4 e). Our results indicate that, because of the superstructures and band hybridization in Bi2212, additional accidental gap nodes or even segments of gapless Fermi surface can be produced besides the usual nodes along the nodal directions in the first Brillouin zone. These should be considered in detecting the gap symmetry of cuprate superconductors 18 , 26 , 27 . Our results also point to the possibility that some unusual superconductors may be designed and produced. As an extreme case, we consider a material that consists of two Fermi surface sheets originating from the initial two degenerate bands due to the interband interaction. As shown in Supplementary Fig. 7 , if the superconducting gap sign is opposite on the two bands, it is possible that the superconducting gap becomes zero on both Fermi surface sheets even though the initial gap is non-zero. This would produce a gapless superconductor with zero superconducting gap on the entire Fermi surface. It is interesting to explore whether such unusual superconductors can be realized both theoretically and experimentally.
In unconventional superconductors, the determination of the gap symmetry, particularly the phase, is both significant and challenging 1 , 12 . In the cuprate superconductors, the phase information of the d -wave gap is obtained by the phase-sensitive experiments based on Josephson tunneling and flux quantization 2 , 9 , 10 . However, these methods were specifically designed for the cuprate superconductors which have relatively simple Fermi surface and d -wave superconducting gap (Fig. 1 a). For the iron-based superconductors that possess multiple Fermi surface sheets and possible unconventional pairing with gap sign changes (Fig. 1 b) 11 – 13 , the conventional phase-sensitive methods based on Josephson tunneling and flux quantization have not been successful in determining the phase of the superconducting gap. The STM measurements have been tried to extract the phase information of the superconducting gap by utilizing quasiparticle interference but this method is limited to only a few materials 14 – 18 . In the present work, we proposed a method to detect the phase of the superconducting gap. It provides direct information of the relative gap sign on the two bands that are studied, regardless of the complexity of the multiple orbital systems. It is a general method that can be applied in various superconductors, including cuprate superconductors, iron-based superconductors, heavy Fermion superconductors and so on. Since ARPES is good at directly determining the anisotropic gap size in the momentum space, with its expanded capability to provide the phase information, it will become a more powerful tool to determine the pairing symmetry of superconductors.
As shown in Fig. 1 and Supplementary Fig. 1 , the applicability of our method depends on the significant difference of the band structures in the superconducting state when the initial superconducting gaps of the two bands have the same or opposite signs. In particular, when the initial superconducting gaps have the opposite sign, some distinct and unusual signatures may appear such as the Fermi momentum shift, the strong Bogoliubov band hybridization and the anomalous gap behaviors. In addition to the relative gap sign, the band structure in the superconducting state is determined by the relative position of the two bare bands, the interband coupling strength and the initial superconducting gap size. The most ideal case to use the method is that the two bare bands are degenerate or adjacent, the two initial gap sizes are the same or very close and the interband coupling is relatively strong. The momentum cut of θ = 33 is close to such an ideal case (the second panel in Fig. 3 b). In less ideal cases when the two bare bands are separated or the two initial gaps are different, the signatures of the relative gap sign become less prominent and can not be identified qualitatively. Careful quantitative analyses are needed to distinguish the relative gap sign. The momentum cuts of θ = 34 and 32 belong to such less ideal cases (the first and third panels in Fig. 3 b). In the cases when the two bare bands are far apart, or the two initial superconducting gaps are significantly different or the interband coupling strength is rather small, the difference induced by the relative gap sign becomes small in the resulted band structures and the method becomes ineffective. Since the effectiveness of the method requires that the two bands are close and have relatively strong interband coupling, it is good for testing the proposed gap structure but is difficult to determine the full momentum-dependence of the phase. The utilization of the method requires an overall understanding of the band structure and superconducting gap structure of the involved two bands.
The Bogoliubov band hybridization between the two bands in Bi2212 or between the two bands from the inner and outer CuO 2 layers in Bi 2 Sr 2 Ca 2 Cu 3 O 10 (Bi2223) 20 , 21 can be universally described by the same two-band model in Equation (1). The extent of the hybridization depends on the separation of the two bands, their interband coupling strength, the superconducting gap size difference on the two bands, and their relative gap sign. In Bi2223, the observed strong Bogoliubov band hybridization between the bands from the inner and outer CuO 2 layers can be well understood by the large superconducting gap size difference of the two bands even though the gaps have the same sign 20 , 21 . In Bi2212, the main bonding band and the main antibonding band have comparable superconducting gap size 28 and the same gap sign as shown in the first quadrant of the Brillouin zone in Fig. 2 a. According to the two-band model, the resulted Bogoliubov band hybridization should be nearly zero. This is consistent with the measured results that no obvious hybridization is observed between the two bands (Supplementary Fig. 5 ). On the other hand, the absence of the Bogoliubov band hybridization between the main bonding band and the main antibonding band indicates that they have the same gap sign. In Bi2212, the main antibonding Fermi surface and the superstructure bonding Fermi surface cross each other in the second quadrant of the Brillouin zone, as shown in Fig. 2 a. Near the crossing region, the main antibonding band and the superstructure bonding band have identical gap size but the opposite gap sign. This gives rise to a dramatic Bogoliubov band hybridization between the two bands as shown in Figs. 2 and 3 . In order to see the obvious Bogoliubov band hybridization effect, the local superconducting gaps should be different on the two bands. In the present case, the gap sign is opposite between the main antibonding band and the superstructure bonding band near the crossing region in the second quadrant.
Our proposed method works well when the two bands are close in the momentum space with a strong interaction between them. It can be used to test whether the two hole-like pockets around the zone center in the iron-based superconductors (Fig. 1 b) have the same or opposite gap sign 29 , 30 . It can also be used to check whether the two electron-like pockets around M (Fig. 1 b) have the same or opposite gap sign, particularly in the iron-based superconductors with only electron pockets 31 – 35 . When the two bands are far away in the momentum space, it is possible to use the method by taking advantage of the band folding from the superstructure or surface reconstruction. In the present work, although the gap sign change occurs on different parts of the Fermi surface, it is detected from the interaction of the main band and the folded band from the superstructures in Bi2212. In the iron-based superconductors like (Ba,K)Fe 2 As 2 , in order to determine the relative gap sign between Γ and M, i.e., whether the gap symmetry is s ± or s ++ 36 – 41 , the 2 × 2 surface reconstruction can be used to fold the bands between the Γ and M points 42 , 43 . In the case that no natural band folding mechanisms are available, it is possible to engineer the band structures to produce hybridization between the initial bands and the superstructure bands such as those realized in twisted graphene 44 – 46 or substrate-controlled film growth 47 , 48 . It should be noted that the key requirement is a perturbative periodic potential that folds the native Fermi surface and thus creates crossing points. This is not far from practical in the age of van-der-Waals heterostructures 49 .
In summary, we have proposed a method to detect superconducting gap sign by ARPES. The method is well tested in the ARPES measurements of Bi2212 with a known d -wave gap symmetry. The gap sign manifests itself in the resulted electronic structures in the superconducting state including the Fermi momentum shift, the Bogoliubov band hybridization and the abnormal superconducting gap behaviors. We have demonstrated that, in addition to measuring the superconducting gap size, ARPES can be phase sensitive and deterministic in pinning down the pairing symmetry that is significant for understanding the superconductivity mechanism of unconventional superconductors.
ARPES measurements were carried out on a vacuum ultraviolet (VUV) laser-based ARPES system equipped with an electron energy analyzer (Scienta Omicron DA30L) 19 , 50 , 51 . The photon energy of the VUV laser is 6.994 eV. The overall energy resolution was set at 1.0 meV. The angular resolution was ~ 0. 3 ∘ , corresponding to a momentum resolution of 0.004 Å −1 with the photon energy of 6.994 eV. The Fermi level is referenced by measuring on the Fermi edge of a clean polycrystalline gold that is electrically connected to the sample. High quality overdoped Bi 2 Sr 2 CaCu 2 O 8+ δ ( T c = 78 K) single crystals were grown by the floating zone method and then annealed in oxygen atmosphere 52 . The T c was measured using a Quantum Design SQUID magnetometer. The sample was cleaved in situ and measured in vacuum with a base pressure better than 3 × 10 −11 mbar.
Supplementary information
Acknowledgements.
This work is supported by the National Key Research and Development Program of China (No. 2021YFA1401800, 2017YFA0302900, 2018YFA0305600, 2018YFA0704200, 2019YFA0308000, 2022YFA1604200 and 2022YFA1403900), the National Natural Science Foundation of China (Grant No. 11888101, 11922414, 11974404 and 12074411), the Strategic Priority Research Program (B) of the Chinese Academy of Sciences (XDB25000000 and XDB33000000), the Innovation Program for Quantum Science and Technology (Grant No. 2021ZD0301800), the Youth Innovation Promotion Association of CAS (Grant No. Y2021006) and Synergetic Extreme Condition User Facility (SECUF). J.M.B. was supported by National Research Foundation (NRF) of Korea through Grants No. NRF-2022R1C1C2008671. The work at Brookhaven was supported by the Office of Basic Energy Sciences, U.S. Department of Energy (DOE) under Contract No de-sc0012704.
Author contributions
X.J.Z., T.X., L.Z., and Q.G. proposed and designed the research. G.D.G., Q.G., and P.A. prepared single crystal. Q.G. carried out the experiment with J.L. and P.A.; Y.Q.C., C.L., Y.W., Q.G., H.T.Y., X.Y.L., C.H.Y., H.C., Z.H.Z., L.Z., G.D.L., F.F.Z., F.Y., S.J.Z., Q.J.P., Z.Y.X., and X.J.Z. contributed to the development and maintenance of Laser ARPES system. Q.G., L.Z., and X.J.Z. analyzed the data. J.M.B., Q.G., and H.Y.C. contributed to model calculations. X.J.Z., L.Z., and Q.G. wrote the paper. All authors discussed the results and commented on the manuscript.
Peer review
Peer review information.
Nature Communications thanks Yu He and Su-Di Chen for their contribution to the peer review of this work. A peer review file is available.
Data availability
Code availability, competing interests.
The authors declare no competing interests.
Publisher’s note Springer Nature remains neutral with regard to jurisdictional claims in published maps and institutional affiliations.
These authors contributed equally: Qiang Gao, Jin Mo Bok, Ping Ai, Jing Liu.
Contributor Information
Lin Zhao, Email: nc.ca.yhpi@oahZL .
Han-Yong Choi, Email: ude.ukks@iohcyh .
X. J. Zhou, Email: nc.ca.yhpi@uohZJX .
The online version contains supplementary material available at 10.1038/s41467-024-48610-9.
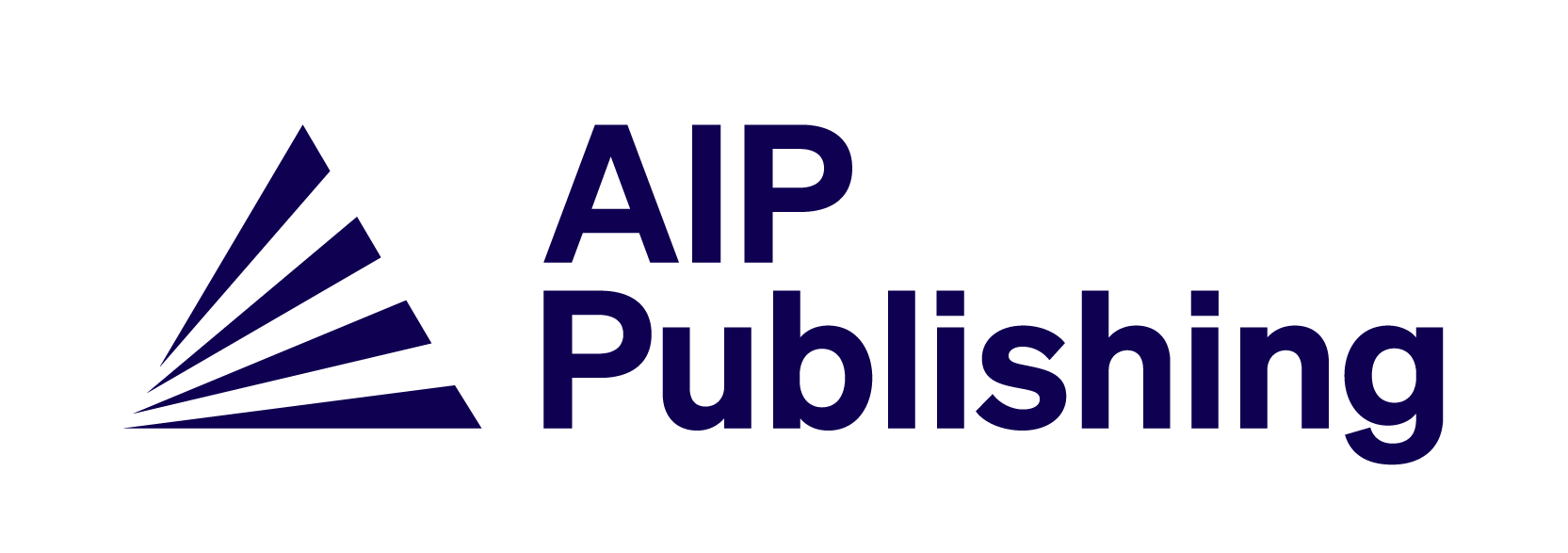
- Previous Article
- Next Article
Superconducting gap and pseudogap
Electronic mail: [email protected]
- Article contents
- Figures & tables
- Supplementary Data
- Peer Review
- Reprints and Permissions
- Cite Icon Cite
- Search Site
Guy Deutscher; Superconducting gap and pseudogap. Low Temp. Phys. 1 June 2006; 32 (6): 566–570. https://doi.org/10.1063/1.2215372
Download citation file:
- Ris (Zotero)
- Reference Manager
The discovery of the pseudogap has been a fundamental advance in uncovering the new physics of the high- T c cuprates, yet its meaning is still far from being clear. In particular, its relation to the superconducting gap remains an object of controversy. While many authors consider that it is a high-temperature precursor of superconductivity, which turns into the superconducting gap at low temperatures, others contend that it is a normal-state property related only indirectly to superconductivity. We review a number of experiments such as single-particle tunneling, Andreev–Saint-James reflections, and others, and conclude that in the underdoped regime there exists considerable evidence for the existence of two distinct energy scales, the superconducting gap and the pseudogap, which appear to merge into one another in overdoped samples.
Citing articles via
Submit your article.
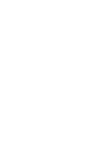
Sign up for alerts
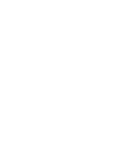
Related Content
- Online ISSN 1090-6517
- Print ISSN 1063-777X
- For Researchers
- For Librarians
- For Advertisers
- Our Publishing Partners
- Physics Today
- Conference Proceedings
- Special Topics

pubs.aip.org
- Privacy Policy
- Terms of Use
Connect with AIP Publishing
This feature is available to subscribers only.
Sign In or Create an Account
- Access through your organization
- Purchase PDF
Article preview
Introduction, section snippets, references (64), cited by (4).

Physica C: Superconductivity
Gaps of cuprate high temperature superconductors, tunnelling spectroscopy, raman scattering, neutron scattering, conclusions and summary, acknowledgements, z. phys. b: condens. matter, rev. mod. phys., rep. prog. phys., phys. rev. b, phys. today, quantum condensates, j. low temp. phys., j. phys. soc. jpn., phys. rev. lett..
- J. Merino, O. Gunnarsson...
Z. Phys. Stat. Sol. B
Phenomenological analysis of the low temperature, structural analysis of the precursor pseudogap in cuprate superconductors, stationary josephson current in junctions involving d-wave superconductors with charge density waves: the temperature dependence and deviations from the law of corresponding states, (re)ba<inf>2</inf>cu<inf>3</inf>o<inf>7−δ</inf> and the roeser-huber formula.
Thank you for visiting nature.com. You are using a browser version with limited support for CSS. To obtain the best experience, we recommend you use a more up to date browser (or turn off compatibility mode in Internet Explorer). In the meantime, to ensure continued support, we are displaying the site without styles and JavaScript.
- View all journals
- Explore content
- About the journal
- Publish with us
- Sign up for alerts
- Published: 18 October 2009
A universal relationship between magnetic resonance and superconducting gap in unconventional superconductors
- E. M. Motoyama 1 &
- M. Greven 2 , 3
Nature Physics volume 5 , pages 873–875 ( 2009 ) Cite this article
4241 Accesses
136 Citations
Metrics details
Superconductivity involves the formation of electron pairs (Cooper pairs) and their condensation into a macroscopic quantum state. In conventional superconductors, such as Nb 3 Ge and elemental Hg, weakly interacting electrons pair through the electron–phonon interaction. In contrast, unconventional superconductivity occurs in correlated-electron materials in which electronic interactions are significant and the pairing mechanism may not be phononic. In the cuprates, the superconductivity arises on doping charge carriers into the copper–oxygen layers of antiferromagnetic Mott insulators 1 . Other examples of unconventional superconductors are the heavy-fermion compounds, which are metals with coupled conduction and localized f -shell electrons 2 , and the recently discovered iron–arsenide superconductors 3 . These unconventional superconductors show a magnetic resonance, a prominent collective spin-1 excitation mode in the superconducting state 4 , 5 , 6 , 7 , 8 . Here we demonstrate the existence of a universal linear relation, E r ∝ 2 Δ , between the magnetic resonance energy ( E r ) and the superconducting pairing gap ( Δ ), which spans two orders of magnitude in energy. This relationship is valid for the three different classes of unconventional superconductors, which range from being close to the Mott-insulating limit to being on the border of itinerant magnetism. As the common excitonic picture of the resonance has not led to such universality, our observation suggests a much deeper connection between antiferromagnetic fluctuations and unconventional superconductivity.
Similar content being viewed by others
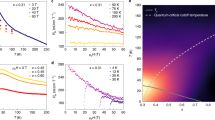
Superconductivity and quantum criticality linked by the Hall effect in a strange metal
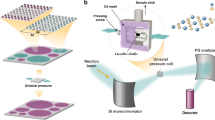
Single-domain stripe order in a high-temperature superconductor
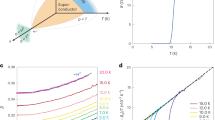
Interplay between superconductivity and the strange-metal state in FeSe
The resonance is a widely discussed feature in the magnetic excitation spectrum of the cuprates. It is a collective mode at a well-defined energy that appears in the superconducting (SC) state at the two-dimensional antiferromagnetic wavevector (π/ a , π/ a ; refs 4 , 5 ). Early inelastic neutron scattering experiments for YBa 2 Cu 3 O 6+ δ (YBCO) revealed a single resonance mode. However, the crystal structure of YBCO contains two nearby Cu–O layers per unit cell, and later experiments revealed at higher energy an even-parity resonance that differs from the previously observed odd-parity mode in its symmetry with respect to the exchange of the two layers 9 , 10 , 11 . The resonance is also observed in neutron scattering measurements of other hole-doped cuprates: double-layer Bi 2 Sr 2 CaCu 2 O 8+ δ (Bi2212; refs 12 , 13 ), and the single-layer systems Tl 2 Ba 2 CuO 6+ δ (Tl2201; ref. 14 ) and HgBa 2 CuO 4+ δ (Hg1201; ref. 15 ), which show only one resonance mode. Investigation of Pr 1− x LaCe x CuO 4+ δ (PLCCO; ref. 16 ) and Nd 2− x Ce x CuO 4+ δ (NCCO; ref. 17 ) suggests that the resonance exists in the electron-doped cuprates as well. Magnetic peaks at the antiferromagnetic zone centre were also found in the SC state of the heavy-fermion superconductors UPd 2 Al 3 (ref. 6 ) and CeCoIn 5 (ref. 7 ), as well as in the iron arsenides Ba 1− x K x Fe 2 As 2 (ref. 8 ; hole doped) and BaFe 2− x Co x As 2 (ref. 18 ; electron doped), indicating that the resonance is a fairly universal feature in the magnetic spectra of unconventional superconductors.
For the cuprates, the mode energy E r of the resonance is commonly compared with the superconducting transition temperature T c . Figure 1 shows that the linear correlation E r =5–6 k B T c is approximately satisfied for the odd-parity mode of the double-layer compounds YBCO and Bi2212, for the resonance in Tl2201 and even for the characteristic energy of the (momentum-integrated) local susceptibility in La 2− x Sr x CuO 4 (LSCO; refs 19 , 20 ), in which no resonance occurs at (π/ a , π/ a ). However, recent experiments reveal a violation of the proportionality between E r and T c for single-layer Hg1201 (ref. 15 ) and for electron-doped NCCO (ref. 17 ). Furthermore, below optimal doping (hole concentration p <0.16), the odd and even resonance energies of the double-layer compounds differ significantly. The average of the two is considerably larger than 6 k B T c for the most underdoped YBCO samples in which both modes have been studied 11 , 21 ( Fig. 1 ).
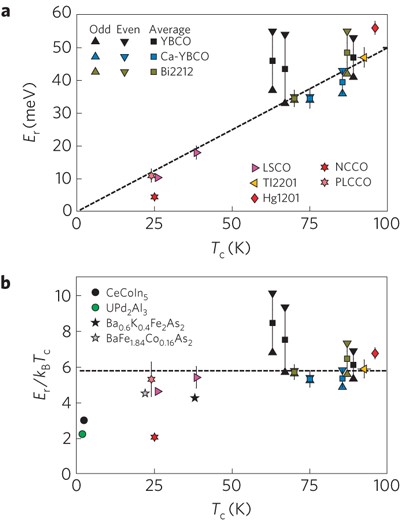
a , E r versus T c for cuprates. For the double-layer hole-doped cuprates (YBCO, Ca-doped YBCO and Bi2212), up (down) triangles indicate odd (even) resonance modes, whereas squares represent the average of the two mode energies 9 , 10 , 11 , 13 , 21 . For LSCO, the resonance is absent and the characteristic energy shown is the maximum in the local magnetic susceptibility, defined as the integral of χ ′′( Q , ω ) over the Brillouin zone 19 , 20 . The interpretation of neutron data for the magnetic resonance in the electron-doped cuprates PLCCO (ref. 16 ) and NCCO (ref. 17 ) is discussed in the Supplementary Information . The dashed line indicates E r =5.8 k B T c . The results for Hg1201 (ref. 15 ), NCCO (ref. 17 ) and the most underdoped YBCO samples 11 , 21 deviate strongly from this relationship. Supplementary Fig. S1 shows the estimated doping dependence of E r for the hole-doped cuprates, including additional results for the odd-parity mode. b , The ratio E r / T c versus T c for all three types of unconventional superconductor. In addition to the results in a , this figure includes those for the heavy-fermion superconductors UPd 2 Al 3 (ref. 6 ) and CeCoIn 5 (ref. 7 ) as well as for the iron arsenides Ba 0.6 K 0.4 Fe 2 As 2 (ref. 8 ) and BaFe 1.84 Co 0.16 As 2 (ref. 18 ). All four show a rather small value of E r / T c . The resonance energy and T c values are summarized in Supplementary Table S1 . Whenever the error bar can be estimated (see Supplementary Information ), it represents one-sigma confidence.
The characteristic energy scale of superconductivity is the SC gap Δ in the low-energy single-particle response. As seen from Fig. 2 , our analysis shows that E r is universally related to Δ rather than to T c . We note that for the hole-doped cuprates the determination of the SC gap is complicated by the existence of multiple energy scales, the deviation of the gap function from a pure d -wave form and spatial inhomogeneity of the gap energy. From photoemission measurements, the SC gap is associated with the gap in the low-energy charge excitations near the nodal region in momentum space, whereas a distinct ‘pseudogap’ is observed in the antinodal region 22 . In the underdoped regime, we estimate the SC gap maximum Δ from the nodal gap Δ 0 determined in recent photoemission experiments. Above optimal doping, we estimate Δ from tunnelling, thermal conductivity and the spatially averaged gap determined in recent scanning tunnelling microscopy measurements. The details of our estimation of Δ in the hole-doped cuprates are found in the Supplementary Information . For the iron arsenides, two SC gaps are observed on different pieces of Fermi surface. We consider the larger of the two, which is associated with nesting bands that are thought to be responsible for the magnetic resonance. For all of the other systems, the SC gap is obtained directly from the literature.
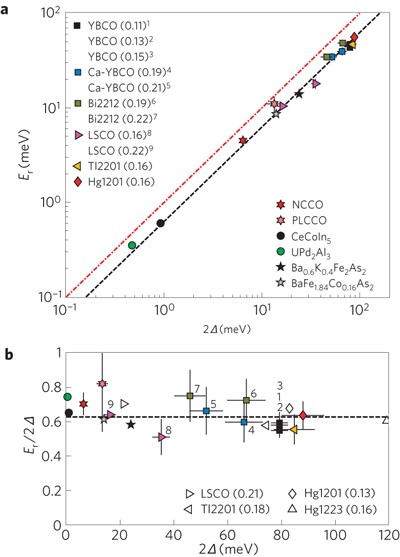
a , The resonance energy E r versus the pair-breaking energy 2 Δ (twice the SC gap). E r of the double-layer cuprates is represented by the average of the even- and odd-parity mode energies. The black dashed line is a fit to all of the data: E r /2 Δ =0.64(4). Unlike the case in Fig. 1 , the results for Hg1201 (ref. 15 ), NCCO (ref. 17 ), and the average mode energy of the most underdoped YBCO samples 11 , 21 all follow this relationship. As a reference, the red dot–dashed line represents E r =2 Δ . Supplementary Fig. S2 shows the resonance energy as a function of 2 Δ on a linear scale. b , The ratio of E r /2 Δ versus 2 Δ . The dashed horizontal line indicates the value E r /2 Δ =0.64. The open symbols indicate the maximum in the bosonic spectral function ( E opt ) extracted from optical spectroscopy for the single-layer cuprates LSCO, Tl2201 and Hg1201, and for triple-layer Hg1223 (ref. 27 ). For the hole-doped cuprates, estimated hole concentrations are shown in parentheses. The 2 Δ values and hole concentrations are obtained as discussed in the Supplementary Information . The characteristic energy and SC gap values are summarized in Supplementary Table S1 (neutron scattering results) and Supplementary Table S2 (optical conductivity results). Whenever the error bar can be estimated (see Supplementary Information ), it represents one-sigma confidence.
We estimate E r /2 Δ =0.64(4) from a fit to the combined result ( Fig. 2 ), which spans two orders of magnitude in energy. A possible connection between the resonance energy and the SC gap has been previously suggested on the basis of observations for the heavy-fermion compound CeCoIn 5 (ref. 7 ), and through a comparison of the odd mode in the double-layer cuprates with the coherent SC gap observed by Andreev reflection 23 . Here we emphasize the universality of this connection across various classes of the unconventional superconductors and, in particular, we arrive at a consistent picture for the entire family of cuprates. For the double-layer systems, which show two resonance modes, we use the mean value of E r in the analysis, rather than only the odd-parity mode.
The magnetic resonance seems to be associated with a SC gap function that undergoes a sign change. This is naturally the case in a Fermi-liquid picture for the d -wave cuprates, because the singularity of the magnetic susceptibility owing to the coherence factor appears at momenta Q =(π/ a ,π/ a ) that satisfy Δ ( q + Q )=− Δ ( q ) (ref. 5 ). We note that, although weak-coupling theory might be appropriate for the very overdoped part of the cuprate phase diagram, it is inadequate at lower doping 1 . The heavy-fermion superconductors considered here also seem to show a d -wave order parameter 2 . On the other hand, the SC gap of the iron arsenides might be nodeless. Nevertheless, the existence of the resonance may be accounted for by the possible antiphase correlation between nesting hole and electron pockets responsible for the magnetic excitations 8 . This is further discussed in the Supplementary Information .
The significance of the linear scaling relation demonstrated in Fig. 2 lies in its simplicity and its universality among different types of unconventional superconductor, which has not been predicted theoretically. In some theoretical models, the resonance is considered to be a π mode, due to staggered d -wave particle–particle charge ±2 excitations, or a magnetic plasmon, as a result of mixing between the spin and charge channels 24 , 25 . The most widely considered theoretical model views the resonance as a spin exciton: a spin-1 particle–hole excitation with momentum Q =(π/ a ,π/ a ) that is bound at an energy below the pair-breaking energy (that is, E r <2 Δ ) (ref. 5 ). The energy difference 2 Δ − E r depends on the specific interactions (for example, spin or charge correlations) stabilizing the bound particle–hole pair and is expected to differ among different classes of superconductors, and for different doping regimes of the hole-doped cuprates. Applied to the hole-doped cuprates, random-phase-approximation approaches to the excitonic picture generally predict the ratio E r /2 Δ to be near one for the very overdoped regime, and to significantly decrease with decreasing hole concentration, rather than to take on a universal value 5 . Such approaches assume weak coupling and are thus expected to break down in the underdoped regime. A recent strong-coupling calculation for the Hubbard model near optimal doping predicts a significant increase of the resonance energy E r with doping 26 , consistent with the behaviour of the odd-parity mode in underdoped YBCO, but inconsistent with the doping independence for the average mode energy of YBCO.
One explanation for the unconventional superconductivity is that it is mediated by magnetic fluctuations. In that case, the SC energy scale Δ would naturally be expected to follow from magnetic energy scales. Such a model implies that the electron–boson spectral function, which contains information about the ‘pairing glue’ for superconductivity, should bear a clear signature of the magnetic spectra. For the cuprates, estimates of the electron–boson spectral function have been obtained by inverting optical spectra 27 , 28 . One approach 27 has revealed a well-defined peak in the SC state at a characteristic energy E opt that agrees remarkably well with E r ( Fig. 2 b), although for the double-layer compounds Bi2212 and YBCO E opt seems to be dominated by the odd-parity resonance mode. For triple-layer Hg1223, for which no neutron data are yet available, E opt ≈72 meV (ref. 27 ) agrees well with the scaling established in Fig. 2 .
Optical spectroscopy is an inherently momentum-integrated probe, and thus the extracted electron–boson spectral function is related to a momentum integral of the magnetic susceptibility χ ′′( Q , ω ) rather than the value at the specific momentum Q =(π/ a ,π/ a ). Furthermore, it has been argued that the magnetic resonance itself has relatively small spectral weight 29 and that magnetic fluctuations throughout an extended momentum region near (π/ a , π/ a ) may be relevant to superconductivity 30 , 31 . Thus the important magnetic energy scale might be the characteristic energy of the local susceptibility χ ′′( ω ). The data seem to be consistent with a scaling between the SC gap and such an energy. For example, for CeCoIn 5 (ref. 7 ), the resonance is very prominent, so that the characteristic energy of χ ′′( ω ) is expected to be close to the value of the resonance energy. For NCCO, the characteristic energies of the peak and local susceptibilities are nearly indistinguishable as well 17 . LSCO is a particularly revealing case: although the main contribution to χ ′′( ω ) below 25 meV does not come from (π/ a , π/ a ), its characteristic energy does indeed scale with the SC gap ( Figs 1 and 2 ).
The observation of a universal connection between the magnetic resonance energy and the SC gap for a wide range of materials seems to indicate that magnetic excitations play an important part in the Cooper-pair formation. To fully understand the connection between the electron–boson spectral function and the magnetic susceptibility, including the resonance, more information from inelastic neutron scattering is needed. Measurements of the doping and temperature dependence of the full magnetic susceptibility of single-layer Hg1201 and of the combined even- and odd-parity excitations in double-layer YBCO and Bi2212 are particularly desirable.
Lee, P. A., Nagaosa, N. & Wen, X. Doping a Mott insulator: Physics of high-temperature superconductivity. Rev. Mod. Phys. 78 , 17–85 (2006).
Article ADS Google Scholar
Goll, G. Unconventional Superconductors: Experimental Investigation of the Order-Parameter Symmetry (Springer, 2005).
Google Scholar
Kamihara, Y. et al. Iron-based layered superconductor La[O1− x F x ]FeAs ( x =0.05–0.12) with T c=26 K. J. Am. Chem. Soc. 130 , 3296–3297 (2008).
Article Google Scholar
Rossat-Mignod, J. et al. Neutron scattering study of the YBa2Cu3O6+ x system. Physica C 185 , 86–92 (1991).
Eschrig, M. The effect of collective spin-1 excitations on electronic spectra in high- T c superconductors. Adv. Phys. 55 , 47–183 (2006).
Sato, N. K. et al. Strong coupling between local moments and superconducting ‘heavy’ electrons in UPd2Al3 . Nature 410 , 340–343 (2001).
Stock, C. et al. Spin resonance in the d -wave superconductor CeCoIn5 . Phys. Rev. Lett. 100 , 087001 (2008).
Christianson, A. D. et al. Unconventional superconductivity in Ba0.6K0.4Fe2As2 from inelastic neutron scattering. Nature 456 , 930–932 (2008).
Pailhès, S. et al. Two resonant magnetic modes in an overdoped high T c superconductor. Phys. Rev. Lett. 91 , 237002 (2003).
Pailhès, S. et al. Resonant magnetic excitations at high energy in superconducting YBa2Cu3O6.85 . Phys. Rev. Lett. 93 , 167001 (2004).
Pailhès, S. et al. Doping dependence of bilayer resonant spin excitations in (Y,Ca)Ba2Cu3O6+ x . Phys. Rev. Lett. 96 , 257001 (2006).
Fong, H. F. et al. Neutron scattering from magnetic excitations in Bi2Sr2CaCu2O8+ δ . Nature 398 , 588–591 (1999).
Capogna, L. et al. Odd and even magnetic resonant modes in highly overdoped Bi2Sr2CaCu2O8+ δ . Phys. Rev. B 75 , 060502R (2007).
He, H. et al. Magnetic resonant mode in the single-layer high-temperature superconductor Tl2Ba2CuO6+ δ . Science 295 , 1045–1047 (2002).
Yu, G. et al. Magnetic resonance in the model high-temperature superconductor HgBa2CuO4+ δ . Preprint at < http://arxiv.org/abs/0810.5759 > (2008).
Wilson, S. D. et al. Resonance in the electron-doped high-transition-temperature superconductor Pr0.88LaCe0.12CuO4− δ . Nature 442 , 59–62 (2006).
Yu, G. et al. Two characteristic energies in the low-energy antiferromagnetic response of the electron-doped high-temperature superconductor Nd2− x Ce x CuO4+ δ . Preprint at < http://arxiv.org/abs/0803.3250 > (2008).
Lumsden, M. D. et al. Two-dimensional resonant magnetic excitation in BaFe1.84Co0.16As2 . Phys. Rev. Lett. 102 , 107005 (2009).
Christensen, N. B. et al. Dispersive excitations in the high-temperature superconductor La2− x Sr x CuO4 . Phys. Rev. Lett. 93 , 147002 (2004).
Lipscombe, O. P. et al. Persistence of high-frequency spin fluctuations in overdoped superconducting La2− x Sr x CuO4 ( x =0.22). Phys. Rev. Lett. 99 , 067002 (2007).
Fong, H. F. et al. Spin susceptibility in underdoped YBa2Cu3O6+ x . Phys. Rev. B 61 , 14773 (2000).
Norman, M. R., Pines, D. & Kallin, C. The pseudogap: Friend or foe of high T c? Adv. Phys. 54 , 715–733 (2005).
Mourachikine, A. The order parameters for pairing and phase coherence in cuprates; the magnetic origin of the coherent gap. The MCS model of high- T c superconductivity. J. Low Temp. Phys. 117 , 401–405 (1999).
Tchernyshyov, O., Norman, M. R. & Chubukov, A. V. Neutron resonance in high- T c superconductors is not the π particle. Phys. Rev. B 63 , 144507 (2001).
Hao, Z. & Chubukov, A. V. Resonance peak in neutron scattering experiments on the cuprates revisited: The case of exciton versus pi-resonance and magnetic plasmon. Phys. Rev. B 79 , 224513 (2009).
Brehm, S., Arrigoni, E., Aichhorn, M. & Hanke, W. Theory of two-particle excitations and the magnetic susceptibility in high- T c cuprate superconductors. Preprint at < http://arxiv.org/abs/0811.0552 > (2008).
Yang, J. et al. Exchange boson dynamics in cuprates: Optical conductivity of HgBa2CuO4+ δ . Phys. Rev. Lett. 102 , 027003 (2009).
van Heumen, E. et al. Optical determination of the relation between the electron–boson coupling function and the critical temperature in high- T c cuprates. Phys. Rev. B 79 , 184512 (2009).
Kee, H.-Y., Kivelson, S. A. & Aeppli, G. Spin-1 neutron resonance peak cannot account for electronic anomalies in the cuprate superconductors. Phys. Rev. Lett. 88 , 257002 (2002).
Kyung, B., Sénéchal, D. & Tremblay, A.-M. S. Pairing dynamics in strongly correlated superconductivity. Preprint at < http://arxiv.org/abs/0812.1228 > (2008).
Abanov, Ar., Chubukov, A. V., Eschrig, M., Norman, M. R. & Schmalian, J. Neutron resonance in the cuprates and its effect on fermionic excitations. Phys. Rev. Lett. 89 , 177002 (2002).
Download references
Acknowledgements
We thank A. V. Chubukov, K. K. Gomes, R.-H. He, S. A. Kivelson and A.-M. Tremblay for comments. This work was supported by the US DOE under contract No DE-AC02-76SF00515 and by the NSF under grant No DMR-0705086.
Author information
Authors and affiliations.
Department of Physics, Stanford University, Stanford, California 94305, USA
G. Yu, Y. Li & E. M. Motoyama
School of Physics and Astronomy, University of Minnesota, Minneapolis, Minnesota 55455, USA
Department of Applied Physics, Stanford University, Stanford, California 94305, USA
You can also search for this author in PubMed Google Scholar
Corresponding author
Correspondence to M. Greven .
Supplementary information
Supplementary information.
Supplementary Information (PDF 768 kb)
Rights and permissions
Reprints and permissions
About this article
Cite this article.
Yu, G., Li, Y., Motoyama, E. et al. A universal relationship between magnetic resonance and superconducting gap in unconventional superconductors. Nature Phys 5 , 873–875 (2009). https://doi.org/10.1038/nphys1426
Download citation
Received : 13 March 2009
Accepted : 04 September 2009
Published : 18 October 2009
Issue Date : December 2009
DOI : https://doi.org/10.1038/nphys1426
Share this article
Anyone you share the following link with will be able to read this content:
Sorry, a shareable link is not currently available for this article.
Provided by the Springer Nature SharedIt content-sharing initiative
This article is cited by
Nodal s± pairing symmetry in an iron-based superconductor with only hole pockets.
- Dingsong Wu
Nature Physics (2024)
Evidence for unconventional superconductivity in twisted trilayer graphene
- Hyunjin Kim
- Youngjoon Choi
- Stevan Nadj-Perge
Nature (2022)
Paramagnons and high-temperature superconductivity in a model family of cuprates
- Lichen Wang
- Guanhong He
Nature Communications (2022)
Resonance from antiferromagnetic spin fluctuations for superconductivity in UTe2
- Chunruo Duan
- R. E. Baumbach
- Pengcheng Dai
Nature (2021)
High-energy magnetic excitations from heavy quasiparticles in CeCu2Si2
npj Quantum Materials (2021)
Quick links
- Explore articles by subject
- Guide to authors
- Editorial policies
Sign up for the Nature Briefing newsletter — what matters in science, free to your inbox daily.

Information
- Author Services
Initiatives
You are accessing a machine-readable page. In order to be human-readable, please install an RSS reader.
All articles published by MDPI are made immediately available worldwide under an open access license. No special permission is required to reuse all or part of the article published by MDPI, including figures and tables. For articles published under an open access Creative Common CC BY license, any part of the article may be reused without permission provided that the original article is clearly cited. For more information, please refer to https://www.mdpi.com/openaccess .
Feature papers represent the most advanced research with significant potential for high impact in the field. A Feature Paper should be a substantial original Article that involves several techniques or approaches, provides an outlook for future research directions and describes possible research applications.
Feature papers are submitted upon individual invitation or recommendation by the scientific editors and must receive positive feedback from the reviewers.
Editor’s Choice articles are based on recommendations by the scientific editors of MDPI journals from around the world. Editors select a small number of articles recently published in the journal that they believe will be particularly interesting to readers, or important in the respective research area. The aim is to provide a snapshot of some of the most exciting work published in the various research areas of the journal.
Original Submission Date Received: .
- Active Journals
- Find a Journal
- Journal Proposal
- Proceedings Series
- For Authors
- For Reviewers
- For Editors
- For Librarians
- For Publishers
- For Societies
- For Conference Organizers
- Open Access Policy
- Institutional Open Access Program
- Special Issues Guidelines
- Editorial Process
- Research and Publication Ethics
- Article Processing Charges
- Testimonials
- Preprints.org
- SciProfiles
- Encyclopedia
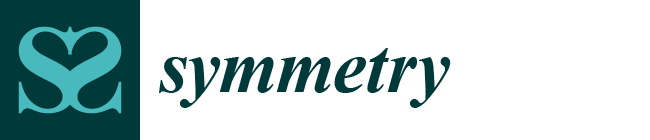
Journal Menu
- Symmetry Home
- Aims & Scope
- Editorial Board
- Reviewer Board
- Topical Advisory Panel
- Instructions for Authors
- Special Issues
- Sections & Collections
- Article Processing Charge
- Indexing & Archiving
- Editor’s Choice Articles
- Most Cited & Viewed
- Journal Statistics
- Journal History
- Journal Awards
- Conferences
- Editorial Office
Journal Browser
- arrow_forward_ios Forthcoming issue arrow_forward_ios Current issue
- Vol. 16 (2024)
- Vol. 15 (2023)
- Vol. 14 (2022)
- Vol. 13 (2021)
- Vol. 12 (2020)
- Vol. 11 (2019)
- Vol. 10 (2018)
- Vol. 9 (2017)
- Vol. 8 (2016)
- Vol. 7 (2015)
- Vol. 6 (2014)
- Vol. 5 (2013)
- Vol. 4 (2012)
- Vol. 3 (2011)
- Vol. 2 (2010)
- Vol. 1 (2009)
Find support for a specific problem in the support section of our website.
Please let us know what you think of our products and services.
Visit our dedicated information section to learn more about MDPI.
Gap Symmetry and Structure of Superconductors
- Print Special Issue Flyer
- Special Issue Editors
Special Issue Information
Benefits of publishing in a special issue.
- Published Papers
A special issue of Symmetry (ISSN 2073-8994). This special issue belongs to the section " Chemistry: Symmetry/Asymmetry ".
Deadline for manuscript submissions: closed (29 February 2020) | Viewed by 31857
Share This Special Issue
Special issue editor.
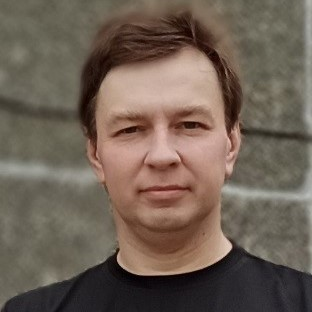
Dear Colleagues,
Symmetry of the gap is the fundamental property of the superconducting state in a material. It is tightly related to the underlying mechanism of Cooper pairing, and, therefore, knowledge of the symmetry put severe constraints on the theories of superconductivity. Even more information can be gained from the particular structure of the gap. The latter term is used to designate the momentum-dependent variation of an order parameter within a given symmetry class. That is, gaps with the same symmetry may have very different structures, such as s+- and s++ states belonging to the same A1g representation in iron pnictides.
The presence of several electronic orbitals in bands near the Fermi level provides both a rich set of properties and complications in revealing the pairing mechanism. Bright examples are the Fe-based pnictides and chalcogenides, sodium cobaltates, and strontium ruthenates. High-Tc cuprates, while sometimes described within single-band models, are also essentially multiband systems allowing to explore a variety of competing ground states. Important feature of these systems is the 'unconventional', non-s-wave, symmetry of the gap.
This Special Issue of Symmetry is devoted to theories and experiments that predict or reveal the gap symmetry and structure of superconductors. Special emphasis is put on the multiband systems with the unconventional order parameter. The scope includes theories of conventional and exotic mechanisms of pairing, and experimental techniques sensitive to the gap symmetry and structure, e.g., penetration depth, thermal conductivity, ARPES, Andreev spectroscopy, inelastic neutron scattering, quasiparticle interference, and Josephson junctions. Contributions can report both a new research and an overview of recent developments.
Manuscripts should be submitted online at www.mdpi.com by registering and logging in to this website . Once you are registered, click here to go to the submission form . Manuscripts can be submitted until the deadline. All submissions that pass pre-check are peer-reviewed. Accepted papers will be published continuously in the journal (as soon as accepted) and will be listed together on the special issue website. Research articles, review articles as well as short communications are invited. For planned papers, a title and short abstract (about 100 words) can be sent to the Editorial Office for announcement on this website.
Submitted manuscripts should not have been published previously, nor be under consideration for publication elsewhere (except conference proceedings papers). All manuscripts are thoroughly refereed through a single-blind peer-review process. A guide for authors and other relevant information for submission of manuscripts is available on the Instructions for Authors page. Symmetry is an international peer-reviewed open access monthly journal published by MDPI.
Please visit the Instructions for Authors page before submitting a manuscript. The Article Processing Charge (APC) for publication in this open access journal is 2400 CHF (Swiss Francs). Submitted papers should be well formatted and use good English. Authors may use MDPI's English editing service prior to publication or during author revisions.
- superconducting gap symmetry and structure
- unconventional superconductors
- iron pnictides and chalcogenides
- high-Tc cuprates
- sodium cobaltates
- strontium ruthenates
- band structure of superconductors
- neutron scattering
- Andreev spectroscopy
- penetration depth
- Ease of navigation: Grouping papers by topic helps scholars navigate broad scope journals more efficiently.
- Greater discoverability: Special Issues support the reach and impact of scientific research. Articles in Special Issues are more discoverable and cited more frequently.
- Expansion of research network: Special Issues facilitate connections among authors, fostering scientific collaborations.
- External promotion: Articles in Special Issues are often promoted through the journal's social media, increasing their visibility.
- e-Book format: Special Issues with more than 10 articles can be published as dedicated e-books, ensuring wide and rapid dissemination.
Further information on MDPI's Special Issue polices can be found here .
Published Papers (5 papers)
Jump to: Review
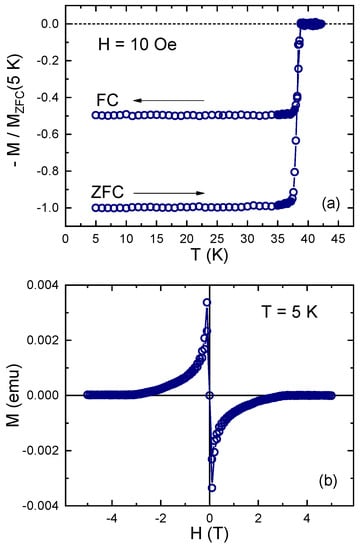
Jump to: Research
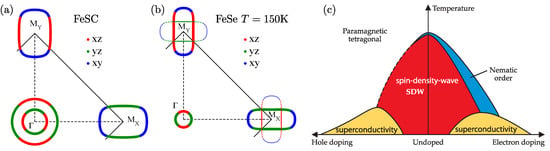
Further Information
Mdpi initiatives, follow mdpi.
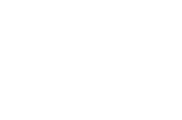
Subscribe to receive issue release notifications and newsletters from MDPI journals

IMAGES
VIDEO
COMMENTS
The superconducting gap symmetry is crucial in understanding the underlying superconductivity mechanism. ... it was obtained later on by the phase-sensitive experiments based on Josephson ...
significant experiments to support this epoch-making theory. One is the isotope effect on the critical temperature, while the other one is the energy gap, later know as superconducting gap, by Michael Tinkham who was recognized with/by Buckley prize. The experimental observation of superconducting gap will be the focus of this paper.
The superconducting gap structure contains important information to understand the pairing mechanism of unconventional superconductivity. ... Our experiments and analyzing technique suggest that ...
Although this marked evolution was identified in several independent experiments, there are no details of the gap evolution to date because of the lack of high-quality single crystals covering the entire K-doping range of the superconducting dome. ... In the optimally doped region, x ≈ 0.35 to 0.4, two effective isotropic superconducting gap ...
The superconducting gap structure is a direct consequence of the mechanism producing the pairing. In phonon-mediated conventional superconductors with a finite on-site pairing ... P. Thalmeier, C. Varma, I. Vekhter, and Y. Yanase for useful discussions. Irradiation experiments were supported by EMIR network, proposal No. 16-0398, 16-9513, and ...
Theory connects the size of the cleaving energy, which is known as the superconducting gap, to the magnitude of the current that tunnels from a normal conductor to a superconductor when the two materials sit on either side of an insulating barrier. ... Experiments reveal the factors that determine the friction between the single-atom-thick ...
Our experiment shows, in the gapless region, ... Pronin, A. V. et al. Direct observation of the superconducting energy gap developing in the conductivity spectra of niobium. Phys. Rev.
An advanced theoretical framework is introduced and examined. Its main idea is to extract properties of the superconducting pairing gap function $Δ(ω)$ in the conventional, nearly localized superconductors. To test the approach, we present an experimentally relevant benchmark model with defined normal and superconducting sectors. The developed reverse engineering framework consists of two ...
compounds [32,66], and thus, calculations and experiments with high accuracy are strongly desired. In this paper we combined state-of-the-art first-principles calculations and tunneling spectroscopy measurements to in-vestigate the superconducting gap functions and mechanisms in Sn 1−xIn xTe. We extended density functional theory for
The signature for paired electrons is thus simple and unambiguous: In a tunneling experiment from a normal metal to a system with bound pairs, the normalized noise should change from S/2I = 1e to S/2I = 2e when the bias is reduced to below the gap energy. Experiments involving conventional superconductors have confirmed the doubling, and even ...
Experiment. We performed high-resolution scanning micro-ARPES experiments on optimally-doped Bi-based high-Tc cuprate Bi 2 Sr 2 CaCu 2 O 8 + ... As our focus is to clarify inhomogeneity in the superconducting gap, we set the region of interest of this work to the antinodal region ...
determine directly the variation of the energy gap on the whole Fermi surface. However, the superconducting gap usually cited in the literature referring to ARPES data is the maximum energy gap, that is, the gap at f › 0 or py2. In tunneling experiments, the gap value is phe-nomenologically defined as the half distance between the ...
More advanced future experiments should build on our current results to fully assess the readiness of Ge for Majorana bound states experiments, such as increasing the induced superconducting gap ...
soft-gap mechanism that summarizes our technical results. For clarity, we would like to emphasize from the very beginning two key points. (1) This is an "intrinsic" mechanism that links the emergence of a smooth in-gap background— the soft gap—to the presence of a metallic lead strongly coupled to the semiconductor nanowire. In addition ...
The superconducting gap symmetry is crucial in understanding the underlying superconductivity mechanism. Angle-resolved photoemission spectroscopy (ARPES) has played a key role in determining the gap symmetry in unconventional superconductors. ... it was obtained later on by the phase-sensitive experiments based on Josephson tunneling and flux ...
The recently discovered superconductor UTe 2 is a promising candidate for spin-triplet superconductors, but the symmetry of the superconducting order parameter remains highly controversial. Here, we determine the superconducting gap structure by the thermal conductivity of ultraclean UTe 2 single crystals. We find that the a-axis thermal conductivity divided by temperature κ/T in zero ...
The point-contact spectra for Nd 1.85 Ce 0.15 CuO 4 showed a well defined superconducting gap structure, which concluded that NCCO is an s -wave superconductor. The size of the superconducting gap (∆) is 3.7 meV and 2∆/ kBTc = 3.9. The Eliashberg function α2F (ω), derived from the tunnel spectra, indicated that Cooper pairing is mediated ...
Γ The superconducting gap sign is marked by blue (positive) and red (negative). b Constant energy contour at the binding energy of 15 meV measured at 15K. The to the Branch1 (BR1, pink line) and ...
Superconducting gap and pseudogap. The discovery of the pseudogap has been a fundamental advance in uncovering the new physics of the high- T c cuprates, yet its meaning is still far from being clear. In particular, its relation to the superconducting gap remains an object of controversy. While many authors consider that it is a high ...
This process is governed by the superconducting gap, which is smaller than the pseudogap. B 2g Raman scattering and neutron scattering can be regarded as inverse AR experiments and thus they also measure the superconducting gap. The difference in AR and GT data is therefore an indication for the pseudogap state being a precursor state to the ...
The low-energy QP excitation spectrum of s ±-wave superconductor, however, is indistinguishable from those of conventional s-wave superconductors because the nodal planes do not exist on the Fermi pockets.Indeed, penetration depth measurement and angle-resolved photoemission spectroscopy (11, 12) suggest that the amplitude of the SC gap is finite all over the Fermi surfaces.
A comprehensive survey of the cuprate, heavy-fermion and iron-based superconductors shows a universal linear relationship between their magnetic resonance energy and superconducting gap. This ...
This Special Issue of Symmetry is devoted to theories and experiments that predict or reveal the gap symmetry and structure of superconductors. Special emphasis is put on the multiband systems with the unconventional order parameter. The scope includes theories of conventional and exotic mechanisms of pairing, and experimental techniques ...