17.1 Understanding Diffraction and Interference
Section learning objectives.
By the end of this section, you will be able to do the following:
- Explain wave behavior of light, including diffraction and interference, including the role of constructive and destructive interference in Young’s single-slit and double-slit experiments
- Perform calculations involving diffraction and interference, in particular the wavelength of light using data from a two-slit interference pattern

Teacher Support
The learning objectives in this section will help your students master the following standards:
- (D) investigate behaviors of waves, including reflection, refraction, diffraction, interference, resonance, and the Doppler effect
Section Key Terms
diffraction | Huygens’s principle | monochromatic | wavefront |
Diffraction and Interference
[BL] Explain constructive and destructive interference graphically on the board.
[OL] Ask students to look closely at a shadow. Ask why the edges are not sharp lines. Explain that this is caused by diffraction, one of the wave properties of electromagnetic radiation. Define the nanometer in relation to other metric length measurements.
[AL] Ask students which, among speed, frequency, and wavelength, stay the same, and which change, when a ray of light travels from one medium to another. Discuss those quantities in terms of colors (wavelengths) of visible light.
We know that visible light is the type of electromagnetic wave to which our eyes responds. As we have seen previously, light obeys the equation
where c = 3.00 × 10 8 c = 3.00 × 10 8 m/s is the speed of light in vacuum, f is the frequency of the electromagnetic wave in Hz (or s –1 ), and λ λ is its wavelength in m. The range of visible wavelengths is approximately 380 to 750 nm. As is true for all waves, light travels in straight lines and acts like a ray when it interacts with objects several times as large as its wavelength. However, when it interacts with smaller objects, it displays its wave characteristics prominently. Interference is the identifying behavior of a wave.
In Figure 17.2 , both the ray and wave characteristics of light can be seen. The laser beam emitted by the observatory represents ray behavior, as it travels in a straight line. Passing a pure, one-wavelength beam through vertical slits with a width close to the wavelength of the beam reveals the wave character of light. Here we see the beam spreading out horizontally into a pattern of bright and dark regions that are caused by systematic constructive and destructive interference. As it is characteristic of wave behavior, interference is observed for water waves, sound waves, and light waves.
That interference is a characteristic of energy propagation by waves is demonstrated more convincingly by water waves. Figure 17.3 shows water waves passing through gaps between some rocks. You can easily see that the gaps are similar in width to the wavelength of the waves and that this causes an interference pattern as the waves pass beyond the gaps. A cross-section across the waves in the foreground would show the crests and troughs characteristic of an interference pattern.
Light has wave characteristics in various media as well as in a vacuum. When light goes from a vacuum to some medium, such as water, its speed and wavelength change, but its frequency, f , remains the same. The speed of light in a medium is v = c / n v = c / n , where n is its index of refraction. If you divide both sides of the equation c = f λ c = f λ by n , you get c / n = v = f λ / n c / n = v = f λ / n . Therefore, v = f λ n v = f λ n , where λ n λ n is the wavelength in a medium, and
where λ λ is the wavelength in vacuum and n is the medium’s index of refraction. It follows that the wavelength of light is smaller in any medium than it is in vacuum. In water, for example, which has n = 1.333, the range of visible wavelengths is (380 nm)/1.333 to (760 nm)/1.333, or λ n = λ n = 285–570 nm. Although wavelengths change while traveling from one medium to another, colors do not, since colors are associated with frequency.
The Dutch scientist Christiaan Huygens (1629–1695) developed a useful technique for determining in detail how and where waves propagate. He used wavefronts , which are the points on a wave’s surface that share the same, constant phase (such as all the points that make up the crest of a water wave). Huygens’s principle states, “Every point on a wavefront is a source of wavelets that spread out in the forward direction at the same speed as the wave itself. The new wavefront is a line tangent to all of the wavelets.”
Figure 17.4 shows how Huygens’s principle is applied. A wavefront is the long edge that moves; for example, the crest or the trough. Each point on the wavefront emits a semicircular wave that moves at the propagation speed v . These are drawn later at a time, t , so that they have moved a distance s = v t s = v t . The new wavefront is a line tangent to the wavelets and is where the wave is located at time t . Huygens’s principle works for all types of waves, including water waves, sound waves, and light waves. It will be useful not only in describing how light waves propagate, but also in how they interfere.
What happens when a wave passes through an opening, such as light shining through an open door into a dark room? For light, you expect to see a sharp shadow of the doorway on the floor of the room, and you expect no light to bend around corners into other parts of the room. When sound passes through a door, you hear it everywhere in the room and, thus, you understand that sound spreads out when passing through such an opening. What is the difference between the behavior of sound waves and light waves in this case? The answer is that the wavelengths that make up the light are very short, so that the light acts like a ray. Sound has wavelengths on the order of the size of the door, and so it bends around corners.
[OL] Discuss the fact that, for a diffraction pattern to be visible, the width of a slit must be roughly the wavelength of the light. Try to give students an idea of the size of visible light wavelengths by noting that a human hair is roughly 100 times wider.
If light passes through smaller openings, often called slits, you can use Huygens’s principle to show that light bends as sound does (see Figure 17.5 ). The bending of a wave around the edges of an opening or an obstacle is called diffraction . Diffraction is a wave characteristic that occurs for all types of waves. If diffraction is observed for a phenomenon, it is evidence that the phenomenon is produced by waves. Thus, the horizontal diffraction of the laser beam after it passes through slits in Figure 17.2 is evidence that light has the properties of a wave.
Once again, water waves present a familiar example of a wave phenomenon that is easy to observe and understand, as shown in Figure 17.6 .
Watch Physics
Single-slit interference.
This video works through the math needed to predict diffraction patterns that are caused by single-slit interference.
Which values of m denote the location of destructive interference in a single-slit diffraction pattern?
- whole integers, excluding zero
- whole integers
- real numbers excluding zero
- real numbers
The fact that Huygens’s principle worked was not considered enough evidence to prove that light is a wave. People were also reluctant to accept light’s wave nature because it contradicted the ideas of Isaac Newton, who was still held in high esteem. The acceptance of the wave character of light came after 1801, when the English physicist and physician Thomas Young (1773–1829) did his now-classic double-slit experiment (see Figure 17.7 ).
When light passes through narrow slits, it is diffracted into semicircular waves, as shown in Figure 17.8 (a). Pure constructive interference occurs where the waves line up crest to crest or trough to trough. Pure destructive interference occurs where they line up crest to trough. The light must fall on a screen and be scattered into our eyes for the pattern to be visible. An analogous pattern for water waves is shown in Figure 17.8 (b). Note that regions of constructive and destructive interference move out from the slits at well-defined angles to the original beam. Those angles depend on wavelength and the distance between the slits, as you will see below.
Virtual Physics
Wave interference.
This simulation demonstrates most of the wave phenomena discussed in this section. First, observe interference between two sources of electromagnetic radiation without adding slits. See how water waves, sound, and light all show interference patterns. Stay with light waves and use only one source. Create diffraction patterns with one slit and then with two. You may have to adjust slit width to see the pattern.
Visually compare the slit width to the wavelength. When do you get the best-defined diffraction pattern?
- when the slit width is larger than the wavelength
- when the slit width is smaller than the wavelength
- when the slit width is comparable to the wavelength
- when the slit width is infinite
Calculations Involving Diffraction and Interference
[BL] The Greek letter θ θ is spelled theta . The Greek letter λ λ is spelled lamda . Both are pronounced the way you would expect from the spelling. The plurals of maximum and minimum are maxima and minima , respectively.
[OL] Explain that monochromatic means one color. Monochromatic also means one frequency . The sine of an angle is the opposite side of a right triangle divided by the hypotenuse. Opposite means opposite the given acute angle. Note that the sign of an angle is always ≥ 1.
The fact that the wavelength of light of one color, or monochromatic light, can be calculated from its two-slit diffraction pattern in Young’s experiments supports the conclusion that light has wave properties. To understand the basis of such calculations, consider how two waves travel from the slits to the screen. Each slit is a different distance from a given point on the screen. Thus different numbers of wavelengths fit into each path. Waves start out from the slits in phase (crest to crest), but they will end up out of phase (crest to trough) at the screen if the paths differ in length by half a wavelength, interfering destructively. If the paths differ by a whole wavelength, then the waves arrive in phase (crest to crest) at the screen, interfering constructively. More generally, if the paths taken by the two waves differ by any half-integral number of wavelengths ( 1 2 λ , 3 2 λ , 5 2 λ , etc .) ( 1 2 λ , 3 2 λ , 5 2 λ , etc .) , then destructive interference occurs. Similarly, if the paths taken by the two waves differ by any integral number of wavelengths ( λ , 2 λ , 3 λ , etc .) ( λ , 2 λ , 3 λ , etc .) , then constructive interference occurs.
Figure 17.9 shows how to determine the path-length difference for waves traveling from two slits to a common point on a screen. If the screen is a large distance away compared with the distance between the slits, then the angle θ θ between the path and a line from the slits perpendicular to the screen (see the figure) is nearly the same for each path. That approximation and simple trigonometry show the length difference, Δ L Δ L , to be d sin θ d sin θ , where d is the distance between the slits,
To obtain constructive interference for a double slit, the path-length difference must be an integral multiple of the wavelength, or
Similarly, to obtain destructive interference for a double slit, the path-length difference must be a half-integral multiple of the wavelength, or
The number m is the order of the interference. For example, m = 4 is fourth-order interference.
Figure 17.10 shows how the intensity of the bands of constructive interference decreases with increasing angle.
Light passing through a single slit forms a diffraction pattern somewhat different from that formed by double slits. Figure 17.11 shows a single-slit diffraction pattern. Note that the central maximum is larger than those on either side, and that the intensity decreases rapidly on either side.
The analysis of single-slit diffraction is illustrated in Figure 17.12 . Assuming the screen is very far away compared with the size of the slit, rays heading toward a common destination are nearly parallel. That approximation allows a series of trigonometric operations that result in the equations for the minima produced by destructive interference.
When rays travel straight ahead, they remain in phase and a central maximum is obtained. However, when rays travel at an angle θ θ relative to the original direction of the beam, each ray travels a different distance to the screen, and they can arrive in or out of phase. Thus, a ray from the center travels a distance λ / 2 λ / 2 farther than the ray from the top edge of the slit, they arrive out of phase, and they interfere destructively. Similarly, for every ray between the top and the center of the slit, there is a ray between the center and the bottom of the slit that travels a distance λ / 2 λ / 2 farther to the common point on the screen, and so interferes destructively. Symmetrically, there will be another minimum at the same angle below the direct ray.
Below we summarize the equations needed for the calculations to follow.
The speed of light in a vacuum, c , the wavelength of the light, λ λ , and its frequency, f , are related as follows.
The wavelength of light in a medium, λ n λ n , compared to its wavelength in a vacuum, λ λ , is given by
To calculate the positions of constructive interference for a double slit, the path-length difference must be an integral multiple, m , of the wavelength. λ λ
where d is the distance between the slits and θ θ is the angle between a line from the slits to the maximum and a line perpendicular to the barrier in which the slits are located. To calculate the positions of destructive interference for a double slit, the path-length difference must be a half-integral multiple of the wavelength:
For a single-slit diffraction pattern, the width of the slit, D , the distance of the first ( m = 1) destructive interference minimum, y , the distance from the slit to the screen, L , and the wavelength, λ λ , are given by
Also, for single-slit diffraction,
where θ θ is the angle between a line from the slit to the minimum and a line perpendicular to the screen, and m is the order of the minimum.
Worked Example
Two-slit interference.
Suppose you pass light from a He-Ne laser through two slits separated by 0.0100 mm, and you find that the third bright line on a screen is formed at an angle of 10.95º relative to the incident beam. What is the wavelength of the light?
The third bright line is due to third-order constructive interference, which means that m = 3. You are given d = 0.0100 mm and θ θ = 10.95º. The wavelength can thus be found using the equation d sin θ = m λ d sin θ = m λ for constructive interference.
The equation is d sin θ = m λ d sin θ = m λ . Solving for the wavelength, λ λ , gives
Substituting known values yields
To three digits, 633 nm is the wavelength of light emitted by the common He-Ne laser. Not by coincidence, this red color is similar to that emitted by neon lights. More important, however, is the fact that interference patterns can be used to measure wavelength. Young did that for visible wavelengths. His analytical technique is still widely used to measure electromagnetic spectra. For a given order, the angle for constructive interference increases with λ λ , so spectra (measurements of intensity versus wavelength) can be obtained.
Single-Slit Diffraction
Visible light of wavelength 550 nm falls on a single slit and produces its second diffraction minimum at an angle of 45.0° relative to the incident direction of the light. What is the width of the slit?
From the given information, and assuming the screen is far away from the slit, you can use the equation D sin θ = m λ D sin θ = m λ to find D .
Quantities given are λ λ = 550 nm, m = 2, and θ 2 θ 2 = 45.0°. Solving the equation D sin θ = m λ D sin θ = m λ for D and substituting known values gives
You see that the slit is narrow (it is only a few times greater than the wavelength of light). That is consistent with the fact that light must interact with an object comparable in size to its wavelength in order to exhibit significant wave effects, such as this single-slit diffraction pattern.
Practice Problems
What is the width of a single slit through which 610-nm orange light passes to form a first diffraction minimum at an angle of 30.0°?
Check Your Understanding
Use these problems to assess student achievement of the section’s learning objectives. If students are struggling with a specific objective, these problems will help identify which and direct students to the relevant topics.
- The wavelength first decreases and then increases.
- The wavelength first increases and then decreases.
- The wavelength increases.
- The wavelength decreases.
- This is a diffraction effect. Your whole body acts as the origin for a new wavefront.
- This is a diffraction effect. Every point on the edge of your shadow acts as the origin for a new wavefront.
- This is a refraction effect. Your whole body acts as the origin for a new wavefront.
- This is a refraction effect. Every point on the edge of your shadow acts as the origin for a new wavefront.
This book may not be used in the training of large language models or otherwise be ingested into large language models or generative AI offerings without OpenStax's permission.
Want to cite, share, or modify this book? This book uses the Creative Commons Attribution License and you must attribute Texas Education Agency (TEA). The original material is available at: https://www.texasgateway.org/book/tea-physics . Changes were made to the original material, including updates to art, structure, and other content updates.
Access for free at https://openstax.org/books/physics/pages/1-introduction
- Authors: Paul Peter Urone, Roger Hinrichs
- Publisher/website: OpenStax
- Book title: Physics
- Publication date: Mar 26, 2020
- Location: Houston, Texas
- Book URL: https://openstax.org/books/physics/pages/1-introduction
- Section URL: https://openstax.org/books/physics/pages/17-1-understanding-diffraction-and-interference
© Jun 7, 2024 Texas Education Agency (TEA). The OpenStax name, OpenStax logo, OpenStax book covers, OpenStax CNX name, and OpenStax CNX logo are not subject to the Creative Commons license and may not be reproduced without the prior and express written consent of Rice University.
Diffraction
Light bends when it passes around an edge or through a slit. This bending is called diffraction. You can easily demonstrate diffraction using a candle or a small bright flashlight bulb and a slit made with two pencils. The diffraction pattern—the pattern of dark and light created when light bends around an edge or edges—shows that light has wavelike properties.
- Two clean new pencils with erasers
- A piece of transparent tape (any thin tape will do)
- A Mini Maglite flashlight ( do not substitute other flashlights ) or a candle with matches or a lighter
- Optional: Pieces of cloth, a feather, plastic diffraction grating, metal screen, a human hair

- Light the candle or, if you are using a Mini Maglite, unscrew the top of the flashlight. The tiny lamp will come on and shine brightly. CAUTION: if you are using an LED Mini Maglite, be sure it is s et to dim before unscrewing the top of the flashlight. Avoid staring directly at the light for a long period of time.
- Wrap one layer of tape around the top of one of the pencils, just below the eraser.
Place the light on a stable surface at least one arm’s length away from you.
Hold up the two pencils, side by side, with the erasers at the top. The tape wrapped around one pencil should keep the pencils slightly apart, forming a thin slit between them, just below the tape. Hold both pencils close to one eye (about 1 inch [2.5 cm] away) and look at the light source through the slit between the pencils. Squeeze the pencils together, making the slit smaller.
Notice that there is a line of light perpendicular to the slit. While looking through the slit, rotate the pencils until they are horizontal, and notice that the line of light becomes vertical.
If you look closely you may see that the line is composed of tiny blobs of light. As you squeeze the slit together, the blobs of light grow larger and spread apart, moving away from the central light source and becoming easier to see. Notice that the blobs have blue and red edges and that the blue edges are closer to the light source.
Stretch a hair tight and hold it about 1 inch (2.5 cm) from your eye. Move the hair until it is between your eye and the light source, and notice that the light is spread into a line of blobs by the hair, just as it was by the slit. Rotate the hair and watch the line of blobs rotate.
Look at the light through a piece of cloth, a feather, a diffraction grating, or a piece of metal screen. Rotate each object while you look through it.
The black bands between the blobs of light show that a wave is associated with the light. The light waves that go through the slit spread out, overlap, and add together, producing the diffraction pattern you see. Where the crest of one wave overlaps with the crest of another wave, the two waves combine to make a bigger wave, and you see a bright blob of light. Where the trough of one wave overlaps with the crest of another wave, the waves cancel each other out, and you see a dark band.
The angle at which the light bends is proportional to the wavelength of the light. Red light, for instance, has a longer wavelength than blue light, so it bends more than blue light does. This different amount of bending gives the blobs their colored edges: blue on the inside, red on the outside.
The narrower the slit, the more the light spreads out. In fact, the angle between two adjacent dark bands in the diffraction pattern is inversely proportional to the width of the slit.
Thin objects, such as a strand of hair, also diffract light. Light that passes around the hair spreads out, overlaps, and produces a diffraction pattern. Cloth and feathers, which are both made up of many smaller, thinner parts, produce complicated diffraction patterns.
In a dimly lit room, look at a Mini Maglite bulb with one eye (a candle will not work). Notice the lines of light radiating out from the light source, like the seeds radiating out from the center of a dandelion.
How can you find the origin of these lines? Rotate the light source and notice that the lines of light do not rotate. Rotate your head and notice that the lines do rotate. Hold your hand or an index card in front of your eye so that it doesn’t quite block your view of the light source (click to enlarge diagram below).
Notice that you still see a full circle of lines radiating out from the light source. The effect actually happens in your eye, as lines of light are spread out onto your retina by imperfections in the tissues of your cornea.
Related Snacks

Related Exhibits

- Why Does Water Expand When It Freezes
- Gold Foil Experiment
- Faraday Cage
- Oil Drop Experiment
- Magnetic Monopole
- Why Do Fireflies Light Up
- Types of Blood Cells With Their Structure, and Functions
- The Main Parts of a Plant With Their Functions
- Parts of a Flower With Their Structure and Functions
- Parts of a Leaf With Their Structure and Functions
- Why Does Ice Float on Water
- Why Does Oil Float on Water
- How Do Clouds Form
- What Causes Lightning
- How are Diamonds Made
- Types of Meteorites
- Types of Volcanoes
- Types of Rocks
Single-Slit Diffraction
Definition: what is the single-slit diffraction.
Single-slit diffraction is the most straightforward experimental setup where diffraction effects can be observed. When light passes through a slit whose width is on the order of the wavelength of light, a distinct diffraction pattern is observed on a screen that is kept at a certain distance from the slit. The intensity is a function of the angle through which the rays bend.
Huygens’ Principle
According to Huygens’ principle, every unobstructed point on a wavefront will act as a source of secondary spherical waves. The new wavefront is the surface tangent to all the secondary spherical waves. Therefore, each part of the slit can be thought of as an emitter of waves.
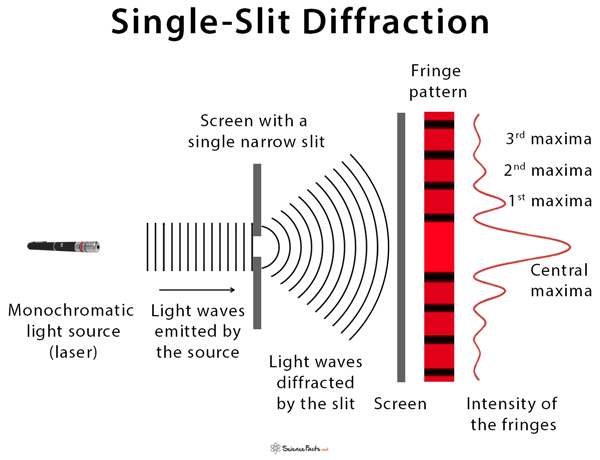
Single Slit Diffraction Pattern
All the waves passing through the slit interfere to produce a diffraction pattern consisting of bright and dark fringes. The bright fringes are due to constructive interference, and the dark areas are due to destructive interference. A number represents the order of the bright and dark fringes. The intensities of the fringes consist of a central maximum surrounded by maxima and minima on its either side. The central maximum is brighter than the other maxima. The maxima rapidly decrease as one moves further from the center.
Single Slit Diffraction Equation
In order to study the diffraction pattern on a screen, the single-slit experiment is employed. Consider a monochromatic source of light that passes through a slit AB of width a as shown in the figure. At point P on the screen, the secondary waves interfere destructively and produce a dark fringe. Let D be the distance between the slit and the screen, and y be the distance between point P and point O, the center of the screen. AC is perpendicular to BP. Let θ be the angle of diffraction, and θ’ is the angle BAC.

We assume that the screen is at a considerable distance from the slit, i.e., D >> a . Hence,
θ = θ’
sin θ ≈ tan θ ≈ θ = y/D
The path difference between the two rays AP and BP is given by,
Δ = BP – AP = BC
In the right-angled triangle BCA,
sin θ’ = sin θ = BC/BA
BC = BA sin θ = a sin θ
Δ = a sin θ
Diffraction Minima
The condition for minima or dark fringe is,
Path difference = integral multiple of wavelength
or, Δ = nλ (n=±1, ±2, ±3, … , etc.)
or, a sin θ = nλ
or, ay/D = nλ
or, y n = nλD/a
This equation gives the distance of the n-th dark fringe from the center.
The fringe width is given by,
β = y n+1 – y n = (n+1)λD/a – nλD/a
or, β = λD/a
Diffraction Maxima
The condition for maxima or bright fringe is,
Path difference = non-integral multiple of wavelength
or, Δ = (n+1/2)λ (n=±1, ±2, ±3, … , etc.)
or, a sin θ = (n+1/2)λ
or, ay/D = (n+1/2)λ
or, y n = (n+1/2)λD/a
The intensity of single-slit diffraction is given by,
I = I 0 [sin (π a sin θ/λ)/( π a sin θ/λ)] 2
Difference Between Single- and Double-Slits Diffraction
Consist of one slit | Consist of two slits |
Waves that originate within the same slit interfere | Slits are so small that each one is considered as a single light source, and the interference of waves originating within the same slit can be neglected |
The fringes are broad and not as sharp as double-slit | The fringes are narrow and sharp |
All bright fringes are visible | Some bright fringes are missing since they suppressed by the minima of a single-slit interference pattern |
Intensity: [sin (π a sin θ/λ)/( π a sin θ/λ)] | Intensity: cos [π d sin θ/λ] [sin (π a sin θ/λ)/( π a sin θ/λ)] |
- Single-Slit Diffraction – Opentextbc.ca
- Single-Slit Diffraction – Labman.phys.utk.edu
- Diffraction from a Single Slit – Animations.physics.unsw.edu.au
- Single-Slit Diffraction – Physics.nus.edu.sg
- Single-Slit Diffraction – Openstax.org
- Fraunhofer Single Slit – Hyperphysics.phy-astr.gsu.edu
- Intensity of Single-Slit Diffraction – Scipp.ucsc.edu
- Interference and Diffraction – Web.mit.edu
Article was last reviewed on Thursday, February 2, 2023
Related articles

Leave a Reply Cancel reply
Your email address will not be published. Required fields are marked *
Save my name, email, and website in this browser for the next time I comment.
Popular Articles

Join our Newsletter
Fill your E-mail Address
Related Worksheets
- Privacy Policy
© 2024 ( Science Facts ). All rights reserved. Reproduction in whole or in part without permission is prohibited.
- Our Education Vision
- Our Solutions

Interference and diffraction of water waves with the Ripple Tank
Article no. P2133500 | Type: Experiments
Description
A set of circular water waves is generated simultaneously and the resulting interference is observed. By increasing the number of interfering circular waves, Huygens' Principle can be verified. With the aid of plane water waves, diffraction phenomena of waves at different obstacles (slit, edge, double-slit etc.) are investigated. In a further experiment, the principle of "phased array antennas" can be demonstrated. To do so, two circular waves are generated to interfere and the resulting interference pattern on varying the phase of one of the circular waves with respect to the other one is observed.
- see and understand the laws that govern wave phenomena
- just add water - compact device offers fast and easy setup
- bright green LED offers qualities even for a demonstration experiment
- Use the comb to generate two circular waves and observe the resulting interference. Increase the number of interfering circular waves up to ten by using all teeth of the comb to demonstrate Huygens' Principle.
- Generate plane water waves and use a barrier to demonstrate diffraction at an edge. Then, form a slit and observe diffraction behind the slit. Repeat this experiment for a double-slit.
- By using the integrated wave generator as well as the external wave generator, generate two circular waves and observe the interference. Vary the phase of the external wave generator and observe the resulting interference pattern to understand the principle of "phased array antennas".
Learning objectives
- Diffraction of water waves
- Interference of waves
- Huygens' Principle
- Principle of "phased arrays antennas"
- Doppler-effect
Scope of delivery
Media / downloads.
- General Terms and Conditions
- Privacy Declaration
- Overview Services
- Webinars & Videos
- Contact Customer Service
- Quality Policy
- Safety in the Classroom
Please note
* Prices subject to VAT.
We only supply companies, institutions and educational facilities. No sales to private individuals.
Please note: To comply with EU regulation 1272/2008 CLP, PHYWE does not sell any chemicals to the general public. We only accept orders from resellers, professional users and research, study and educational institutions.
Our website uses cookies. Some of them are essential, others help us improve this website and your user experience. You can find further information about our use of cookies and your rights as a user in our Privacy policy and our Legal disclosure .
Please enter a name under which your shopping cart should be saved. You can find your saved shopping carts in the My Account section.
Shopping Cart Name
- Testimonials
- Publications
- Class Schedule
- Terms and Conditions
- JC Syllabus
- IP/Secondary
Diffraction of Waves: Single Slit & Multiple Slit Diffraction
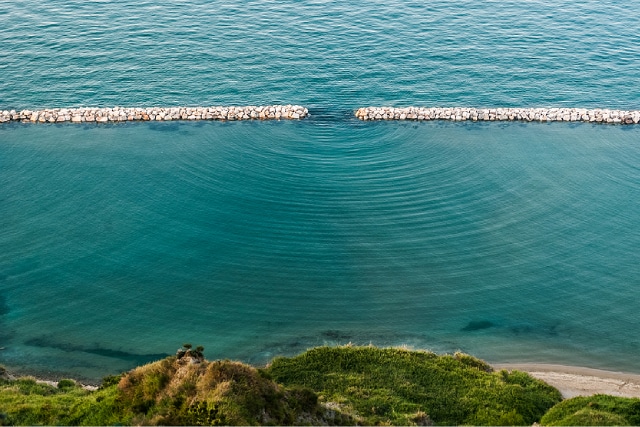
Forming a shadow of an object or the bending of light at the corners of the door are real-life examples of diffraction. Read on to learn more about the diffraction of waves!
What is diffraction?
When waves come into contact with an obstacle or go through a slit, it exhibits a behaviour called diffraction. Diffraction is defined as the bending of waves around the corners or opening of an obstacle, and it can happen to any forms of waves, including water waves, light waves, and sound waves .
Diffraction can be demonstrated by placing an obstacle in a ripple tank and observing the water wave’s path. You will notice that the waves that pass the object are disturbed. The amount of diffraction that occurs increases when the wavelength of the wave increases.
For example, water wave diffraction is observed when waves bend around small boats, and the water behind them is disturbed. However, the same waves are unable to diffract around bigger boats since their wavelength is smaller than the boat.
Now that you have a basic understanding of what diffraction is, let’s find out a little more about single slit and multiple slit diffraction.
The single slit experiment
The bending phenomenon of diffraction can be observed in a single-slit diffraction experiment where the wave from a source interferes with itself and produces a distinctive pattern called the diffraction pattern. In single slit diffraction, the diffraction pattern is determined by the wavelength and by the length of the slit. Decreasing the size of the opening can increase the amount of diffraction. To get true diffraction, the slit has to be the size of lambda, the size of the wavelength.
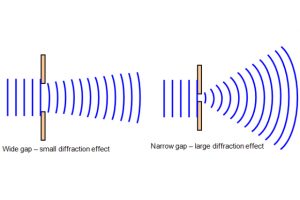
Image Credit: https://sites.google.com/site/thepropertiesofwaves/diffra
Multiple slit diffraction
When waves pass through a large number of evenly spaced parallel slits, diffraction grating occurs where an interference pattern is created. For light, diffraction grating works both for light transmission and reflection. The pattern of interference consists of bright and dark fringes that are sequential. The dark fringes are the product of destructive interference, and the bright fringes are the result of constructive interference.
Some everyday examples where we see multiple slit diffraction of light are surfaces like the back of a CD, or minerals like pearl, opal, or the inner surface of oyster shells. These appear to have different combinations of colours when viewed from different angles, a direct manifestation of multiple slit diffraction.
Comparing single slit and multiple slit diffraction
The diffraction pattern in the single slit experiment is very much like the multiple slit interference, except instead of multiple slits, the wavefront itself splits into a bunch of adjacent point sources that interact with each other.
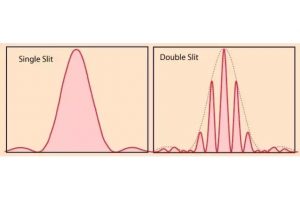
Image Credit: https://www.quora.com/What-is-the-difference-between-single-slit-and-double-slit-diffraction
What happens when two waves meet?
Wave interference is a phenomenon that happens when two waves meet while travelling along with the same medium. An interesting pattern is formed in a ripple tank when there is an interference of two sources of periodic and concentric waves of the same frequency. The light waves from two sources interfere whenever the thick line from one light source meets another thick line from the other sources. The same thing happens with the thin lines. The diagram below shows the pattern of interference caused by two periodic disturbances.
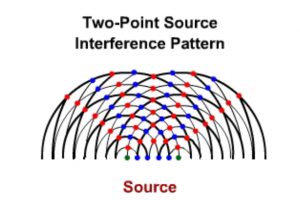
Image Credit: http://aventalearning.com/content168staging/2008Physics/SectionB/unit7/html/section_4_page_24.html
Diffraction is a phenomenon that occurs everywhere around us. Now that you have a better understanding of it, try noticing some during your day! If you found this interesting, sign up for A level physics tuition classes to learn more! We also provide O level physics tuition for those who have their exams coming.

TuitionPhysics
Copyright: Best Physics Tuition™ Centre. All Rights Reserved | User Sitemap
We think you are located in Russian Federation . Is this correct?
- Yes, I reside in Russian Federation
- Change country/curriculum
We use this information to present the correct curriculum and to personalise content to better meet the needs of our users.
6.4 Diffraction
6.4 Diffraction (ESBNH)
One of the most interesting, and also very useful, properties of waves is diffraction .
Diffraction is the ability of a wave to spread out in wavefronts as the wave passes through a small aperture or around a sharp edge.
For example, if two rooms are connected by an open doorway and a sound is produced in a remote corner of one of them, a person in the other room will hear the sound as if it originated at the doorway.

As far as the second room is concerned, the vibrating air in the doorway is the source of the sound.
This means that when waves move through small holes they appear to bend around the sides because there are not enough points on the wavefront to form another straight wavefront. This is bending round the sides we call diffraction .
Diffraction effects are more clear for water waves with longer wavelengths. Diffraction can be demonstrated by placing small barriers and obstacles in a ripple tank and observing the path of the water waves as they encounter the obstacles. The waves are seen to pass around the barrier into the regions behind it; subsequently the water behind the barrier is disturbed. The amount of diffraction (the sharpness of the bending) increases with increasing wavelength and decreases with decreasing wavelength. In fact, when the wavelength of the waves are smaller than the obstacle, no noticeable diffraction occurs.
This experiment demonstrates diffraction using water waves in a ripple tank. You can also demonstrate diffraction using a single slit and a light source with coloured filters.
Diffraction
Water waves in a ripple tank can be used to demonstrate diffraction and interference.
Turn on the wave generator so that it produces waves with a high frequency (short wavelength).
Place a few obstacles, one at a time, (e.g. a brick or a ruler) in the ripple tank. What happens to the wavefronts as they propagate near/past the obstacles? Draw your observations.
How does the diffraction change when you change the size of the object?
Now turn down the frequency of the wave generator so that it produces waves with longer wavelengths.
Place the same obstacles in the ripple tank (one at a time). What happens to the wavefronts as they propagate near/past the obstacles? Draw your observations.
How does the diffraction change from the higher frequency case?
Remove all obstacles from the ripple tank and insert a second wave generator. Turn on both generators so that they start at the same time and have the same frequency.
What do you notice when the two sets of wavefronts meet each other?
Can you identify regions of constructive and destructive interference?
Now turn on the generators so that they are out of phase (i.e. start them so that they do not make waves at exactly the same time).

17.1 Understanding Diffraction and Interference
Learning objectives.
By the end of this section, you will be able to do the following:
- Explain wave behavior of light, including diffraction and interference, including the role of constructive and destructive interference in Young’s single-slit and double-slit experiments
- Perform calculations involving diffraction and interference, in particular the wavelength of light using data from a two-slit interference pattern
diffraction | Huygens’s principle | monochromatic |
wavefront |
Diffraction and Interference
We know that visible light is the type of electromagnetic wave to which our eyes responds. As we have seen previously, light obeys the equation
where c = 3.00 × 10 8 c = 3.00 × 10 8 m/s is the speed of light in vacuum, f is the frequency of the electromagnetic wave in Hz (or s –1 ), and λ λ is its wavelength in m. The range of visible wavelengths is approximately 380 to 750 nm. As is true for all waves, light travels in straight lines and acts like a ray when it interacts with objects several times as large as its wavelength. However, when it interacts with smaller objects, it displays its wave characteristics prominently. Interference is the identifying behavior of a wave.
In Figure 17.2 , both the ray and wave characteristics of light can be seen. The laser beam emitted by the observatory represents ray behavior, as it travels in a straight line. Passing a pure, one-wavelength beam through vertical slits with a width close to the wavelength of the beam reveals the wave character of light. Here we see the beam spreading out horizontally into a pattern of bright and dark regions that are caused by systematic constructive and destructive interference. As it is characteristic of wave behavior, interference is observed for water waves, sound waves, and light waves.
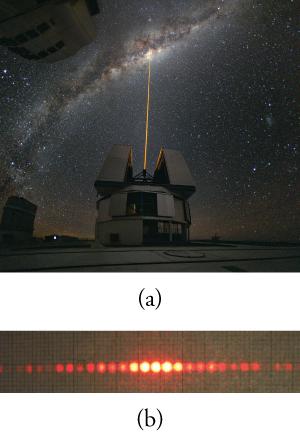
That interference is a characteristic of energy propagation by waves is demonstrated more convincingly by water waves. Figure 17.3 shows water waves passing through gaps between some rocks. You can easily see that the gaps are similar in width to the wavelength of the waves and that this causes an interference pattern as the waves pass beyond the gaps. A cross-section across the waves in the foreground would show the crests and troughs characteristic of an interference pattern.
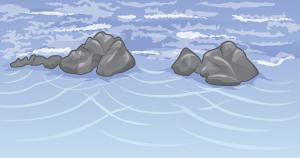
Light has wave characteristics in various media as well as in a vacuum. When light goes from a vacuum to some medium, such as water, its speed and wavelength change, but its frequency, f , remains the same. The speed of light in a medium is v = c / n v = c / n , where n is its index of refraction. If you divide both sides of the equation c = f λ c = f λ by n , you get c / n = v = f λ / n c / n = v = f λ / n . Therefore, v = f λ n v = f λ n , where λ n λ n is the wavelength in a medium, and
where λ λ is the wavelength in vacuum and n is the medium’s index of refraction. It follows that the wavelength of light is smaller in any medium than it is in vacuum. In water, for example, which has n = 1.333, the range of visible wavelengths is (380 nm)/1.333 to (760 nm)/1.333, or λ n = λ n = 285–570 nm. Although wavelengths change while traveling from one medium to another, colors do not, since colors are associated with frequency.
The Dutch scientist Christiaan Huygens (1629–1695) developed a useful technique for determining in detail how and where waves propagate. He used wavefronts , which are the points on a wave’s surface that share the same, constant phase (such as all the points that make up the crest of a water wave). Huygens’s principle states, “Every point on a wavefront is a source of wavelets that spread out in the forward direction at the same speed as the wave itself. The new wavefront is a line tangent to all of the wavelets.”
Figure 17.4 shows how Huygens’s principle is applied. A wavefront is the long edge that moves; for example, the crest or the trough. Each point on the wavefront emits a semicircular wave that moves at the propagation speed v . These are drawn later at a time, t , so that they have moved a distance s = v t s = v t . The new wavefront is a line tangent to the wavelets and is where the wave is located at time t . Huygens’s principle works for all types of waves, including water waves, sound waves, and light waves. It will be useful not only in describing how light waves propagate, but also in how they interfere.
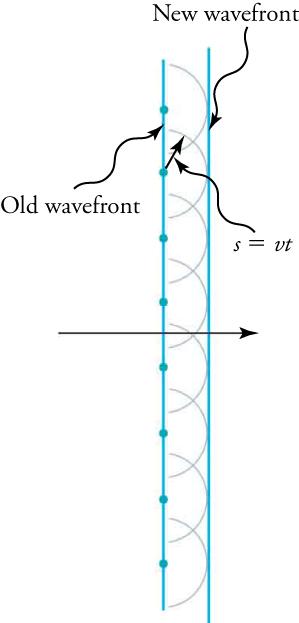
What happens when a wave passes through an opening, such as light shining through an open door into a dark room? For light, you expect to see a sharp shadow of the doorway on the floor of the room, and you expect no light to bend around corners into other parts of the room. When sound passes through a door, you hear it everywhere in the room and, thus, you understand that sound spreads out when passing through such an opening. What is the difference between the behavior of sound waves and light waves in this case? The answer is that the wavelengths that make up the light are very short, so that the light acts like a ray. Sound has wavelengths on the order of the size of the door, and so it bends around corners.
If light passes through smaller openings, often called slits, you can use Huygens’s principle to show that light bends as sound does (see Figure 17.5 ). The bending of a wave around the edges of an opening or an obstacle is called diffraction . Diffraction is a wave characteristic that occurs for all types of waves. If diffraction is observed for a phenomenon, it is evidence that the phenomenon is produced by waves. Thus, the horizontal diffraction of the laser beam after it passes through slits in Figure 17.2 is evidence that light has the properties of a wave.
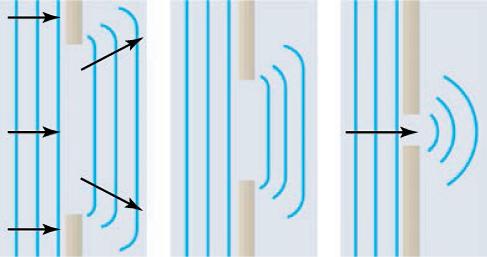
Once again, water waves present a familiar example of a wave phenomenon that is easy to observe and understand, as shown in Figure 17.6 .
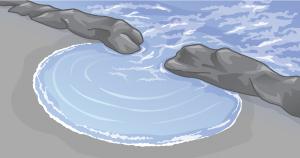
Watch Physics
Single-slit interference.
This video works through the math needed to predict diffraction patterns that are caused by single-slit interference.
Which values of m denote the location of destructive interference in a single-slit diffraction pattern?
- whole integers, excluding zero
- whole integers
- real numbers excluding zero
- real numbers
The fact that Huygens’s principle worked was not considered enough evidence to prove that light is a wave. People were also reluctant to accept light’s wave nature because it contradicted the ideas of Isaac Newton, who was still held in high esteem. The acceptance of the wave character of light came after 1801, when the English physicist and physician Thomas Young (1773–1829) did his now-classic double-slit experiment (see Figure 17.7 ).
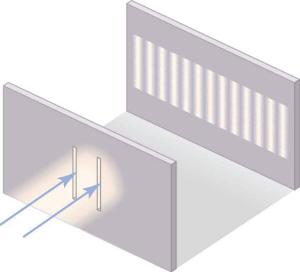
When light passes through narrow slits, it is diffracted into semicircular waves, as shown in Figure 17.8 (a). Pure constructive interference occurs where the waves line up crest to crest or trough to trough. Pure destructive interference occurs where they line up crest to trough. The light must fall on a screen and be scattered into our eyes for the pattern to be visible. An analogous pattern for water waves is shown in Figure 17.8 (b). Note that regions of constructive and destructive interference move out from the slits at well-defined angles to the original beam. Those angles depend on wavelength and the distance between the slits, as you will see below.
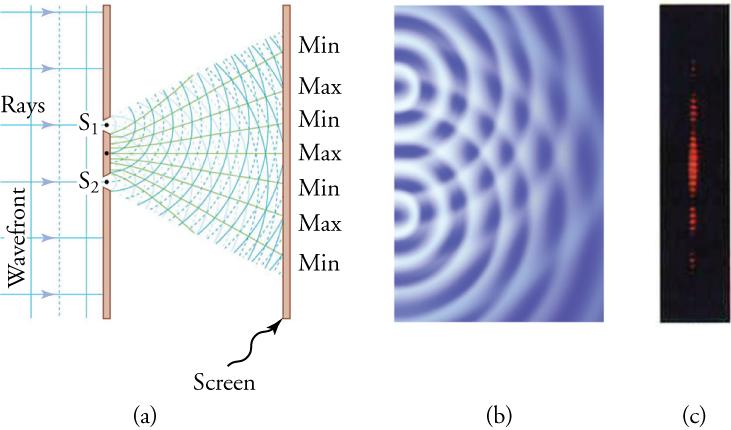
Virtual Physics
Wave interference.
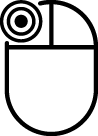
This simulation demonstrates most of the wave phenomena discussed in this section. First, observe interference between two sources of electromagnetic radiation without adding slits. See how water waves, sound, and light all show interference patterns. Stay with light waves and use only one source. Create diffraction patterns with one slit and then with two. You may have to adjust slit width to see the pattern.
Visually compare the slit width to the wavelength. When do you get the best-defined diffraction pattern?
- when the slit width is larger than the wavelength
- when the slit width is smaller than the wavelength
- when the slit width is comparable to the wavelength
- when the slit width is infinite
Calculations Involving Diffraction and Interference
The fact that the wavelength of light of one color, or monochromatic light, can be calculated from its two-slit diffraction pattern in Young’s experiments supports the conclusion that light has wave properties. To understand the basis of such calculations, consider how two waves travel from the slits to the screen. Each slit is a different distance from a given point on the screen. Thus different numbers of wavelengths fit into each path. Waves start out from the slits in phase (crest to crest), but they will end up out of phase (crest to trough) at the screen if the paths differ in length by half a wavelength, interfering destructively. If the paths differ by a whole wavelength, then the waves arrive in phase (crest to crest) at the screen, interfering constructively. More generally, if the paths taken by the two waves differ by any half-integral number of wavelengths ( 1 2 λ , 3 2 λ , 5 2 λ , etc .) ( 1 2 λ , 3 2 λ , 5 2 λ , etc .) , then destructive interference occurs. Similarly, if the paths taken by the two waves differ by any integral number of wavelengths ( λ , 2 λ , 3 λ , etc .) ( λ , 2 λ , 3 λ , etc .) , then constructive interference occurs.
Figure 17.10 shows how to determine the path-length difference for waves traveling from two slits to a common point on a screen. If the screen is a large distance away compared with the distance between the slits, then the angle θ θ between the path and a line from the slits perpendicular to the screen (see the figure) is nearly the same for each path. That approximation and simple trigonometry show the length difference, Δ L Δ L , to be d sin θ d sin θ , where d is the distance between the slits,
To obtain constructive interference for a double slit, the path-length difference must be an integral multiple of the wavelength, or
Similarly, to obtain destructive interference for a double slit, the path-length difference must be a half-integral multiple of the wavelength, or
The number m is the order of the interference. For example, m = 4 is fourth-order interference.
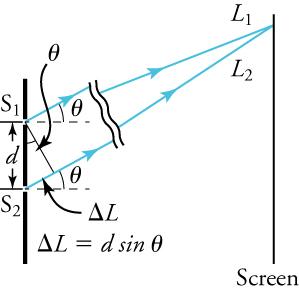
Figure 17.11 shows how the intensity of the bands of constructive interference decreases with increasing angle.
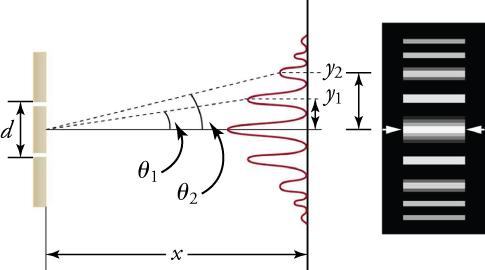
Light passing through a single slit forms a diffraction pattern somewhat different from that formed by double slits. Figure 17.12 shows a single-slit diffraction pattern. Note that the central maximum is larger than those on either side, and that the intensity decreases rapidly on either side.
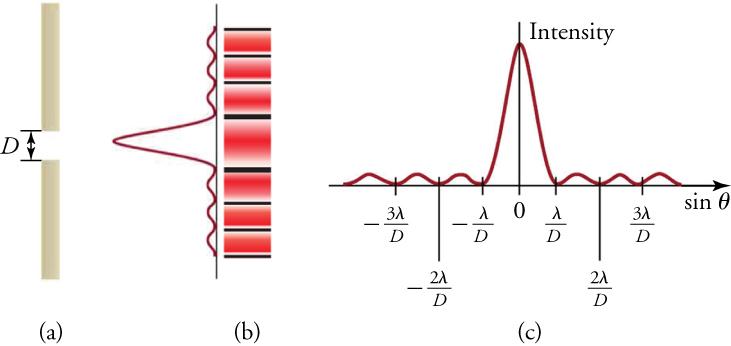
The analysis of single-slit diffraction is illustrated in Figure 17.13 . Assuming the screen is very far away compared with the size of the slit, rays heading toward a common destination are nearly parallel. That approximation allows a series of trigonometric operations that result in the equations for the minima produced by destructive interference.
When rays travel straight ahead, they remain in phase and a central maximum is obtained. However, when rays travel at an angle θ θ relative to the original direction of the beam, each ray travels a different distance to the screen, and they can arrive in or out of phase. Thus, a ray from the center travels a distance λ / 2 λ / 2 farther than the ray from the top edge of the slit, they arrive out of phase, and they interfere destructively. Similarly, for every ray between the top and the center of the slit, there is a ray between the center and the bottom of the slit that travels a distance λ / 2 λ / 2 farther to the common point on the screen, and so interferes destructively. Symmetrically, there will be another minimum at the same angle below the direct ray.
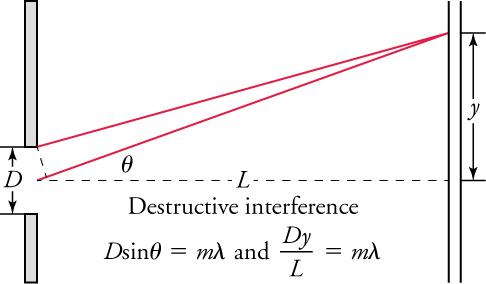
Below we summarize the equations needed for the calculations to follow.
The speed of light in a vacuum, c , the wavelength of the light, λ λ , and its frequency, f , are related as follows.
The wavelength of light in a medium, λ n λ n , compared to its wavelength in a vacuum, λ λ , is given by
To calculate the positions of constructive interference for a double slit, the path-length difference must be an integral multiple, m , of the wavelength. λ λ
where d is the distance between the slits and θ θ is the angle between a line from the slits to the maximum and a line perpendicular to the barrier in which the slits are located. To calculate the positions of destructive interference for a double slit, the path-length difference must be a half-integral multiple of the wavelength:
For a single-slit diffraction pattern, the width of the slit, D , the distance of the first ( m = 1) destructive interference minimum, y , the distance from the slit to the screen, L , and the wavelength, λ λ , are given by
Also, for single-slit diffraction,
where θ θ is the angle between a line from the slit to the minimum and a line perpendicular to the screen, and m is the order of the minimum.
Worked Example
Two-slit interference.
Suppose you pass light from a He-Ne laser through two slits separated by 0.0100 mm, and you find that the third bright line on a screen is formed at an angle of 10.95º relative to the incident beam. What is the wavelength of the light?
The third bright line is due to third-order constructive interference, which means that m = 3. You are given d = 0.0100 mm and θ θ = 10.95º. The wavelength can thus be found using the equation d sin θ = m λ d sin θ = m λ for constructive interference.
The equation is d sin θ = m λ d sin θ = m λ . Solving for the wavelength, λ λ , gives
Substituting known values yields
To three digits, 633 nm is the wavelength of light emitted by the common He-Ne laser. Not by coincidence, this red color is similar to that emitted by neon lights. More important, however, is the fact that interference patterns can be used to measure wavelength. Young did that for visible wavelengths. His analytical technique is still widely used to measure electromagnetic spectra. For a given order, the angle for constructive interference increases with λ λ , so spectra (measurements of intensity versus wavelength) can be obtained.
Single-Slit Diffraction
Visible light of wavelength 550 nm falls on a single slit and produces its second diffraction minimum at an angle of 45.0° relative to the incident direction of the light. What is the width of the slit?
From the given information, and assuming the screen is far away from the slit, you can use the equation D sin θ = m λ D sin θ = m λ to find D .
Quantities given are λ λ = 550 nm, m = 2, and θ 2 θ 2 = 45.0°. Solving the equation D sin θ = m λ D sin θ = m λ for D and substituting known values gives
You see that the slit is narrow (it is only a few times greater than the wavelength of light). That is consistent with the fact that light must interact with an object comparable in size to its wavelength in order to exhibit significant wave effects, such as this single-slit diffraction pattern.
Practice Problems
What is the width of a single slit through which 610-nm orange light passes to form a first diffraction minimum at an angle of 30.0°?
Check Your Understanding
- The wavelength first decreases and then increases.
- The wavelength first increases and then decreases.
- The wavelength increases.
- The wavelength decreases.
- This is a diffraction effect. Your whole body acts as the origin for a new wavefront.
- This is a diffraction effect. Every point on the edge of your shadow acts as the origin for a new wavefront.
- This is a refraction effect. Your whole body acts as the origin for a new wavefront.
- This is a refraction effect. Every point on the edge of your shadow acts as the origin for a new wavefront.
Copy and paste the link code above.
Related Items
- Skip to main content
- Skip to primary sidebar

Water Refraction Science Experiment
This water refraction science experiment has such a "wow factor" and is so quick and easy I can't believe my kids and I haven't done it before. It would fit right in with our DIY science camp series , too. Best of all, there is almost no set up, but once I showed it to the kids, they experimented on their own and debated the reasons for the results. You have the option of setting up the science experiment as we did, or doing it right now with the glass of water sitting next to you!

What you need:
- Jar or glass
- A paper with a design on it. If you wish, you can download and print our printable . It is two pages and includes the colored bars and two arrows.

Instructions:
Place a jar or glass about 6 inches in front of the colored bars or arrows. Pour in the water. What happens?
Watch the video!
To make it more fun:
Ask your kids to keep their eyes on the bars/arrows as you slowly pour the water into the jar.
I told my kids I had a magic liquid. I'm pretty sure my 6 year old believed me, but my 10 year old unconvincingly said, "It's water, mom." But then after I performed the experiment, he said, "Now try it with water." Ha! So I guess I did fool him.

Explorations:
- Does it make a difference how close the water is to the paper?
- Does it make a difference if the jar is square or round? What about the size of the jar or glass?
- Draw a diagram of what you think is happening to the light rays. (See explanation below)
- Super nerdy kids ( I say that with love ) can learn more about refraction of light here .
The science behind the water refraction experiment:
Refraction is the bending of light. In this case, light traveled from the air, through the front of the glass jar, through the water, through the back of the glass jar, and then back through the air, before hitting the picture. Whenever light passes from one medium into another, it refracts.
In addition, the water acts as a magnifying glass, which bends the light toward the center. The light comes together at the focal point and beyond the focal point the image looks reversed because the light that was on the right is now on the left, and vice versa. Clear as mud? ( Note: I am not a science teacher and if you would like to correct or add to my explanation in the comments, I welcome it! Update: someone did! Read the comments for more scientific explanation of the refraction phenomena. )
Want another cool and magical water experiment?
- Find out how to make a coin jump from a bottle .
- Or find more fun indoor water activities for kids .

Reader Interactions
Maria Dermitzaki says
February 12, 2017 at 3:25 pm
I would like to receive mails
February 13, 2017 at 9:44 am
You can sign up here: https://www.whatdowedoallday.com/newsletter-sign
Eliana Nevarez says
February 04, 2018 at 10:59 pm
we filled the glass half with water and put the arrow behind the glass and when we moved the arrow to a particular distance behind the glass it makes the arrow look like its going the other way. When light passes from one material to another, it can bend or refract. In the third experiment before hitting the arrow light traveled from the air, through the glass, through the water, through the back of the glass, and then back through the air. Anytime that light passes from one thing into another, it refracts. When light went through the glass the light bent toward the center. That’s where the light all came together this is called the focal point, but beyond the focal point the image reversed because the light rays that were bent pass each other and the light that was on the right side is now on the left and the left on the right and that is what makes the arrow looks reversed.
Sauli Jämsä says
March 30, 2020 at 7:48 am
You should be talking about light traveling FROM the picture TO your eyes.
June 03, 2020 at 9:41 am
Light doesn't travel, it's just waves.
September 19, 2020 at 5:54 am
What is the prinsipal for this experiment
ayushi says
May 12, 2021 at 3:16 am
prinicipal - refraction of light.
person says
February 10, 2022 at 2:15 pm
you spelt principal wrong
February 10, 2022 at 2:16 pm
ranji spelt principal wrong*
February 22, 2021 at 3:58 am
It will just be same if the glass is not round?
February 22, 2021 at 3:15 pm
Might be a fun experiment to try it and find out. 😉
Ms. Right says
January 14, 2023 at 12:04 pm
Everyone spelled it wrong. Principal = the leader of a school. Principle = theory of reasoning
Leave a Reply Cancel reply
Your email address will not be published. Required fields are marked *
This site uses Akismet to reduce spam. Learn how your comment data is processed .
Look! We're Learning!
Early Learning. Happy Teaching.
40 Simple Water Science Experiments for Kids
April 16, 2018 by Selena Robinson Leave a Comment
Sharing is caring!
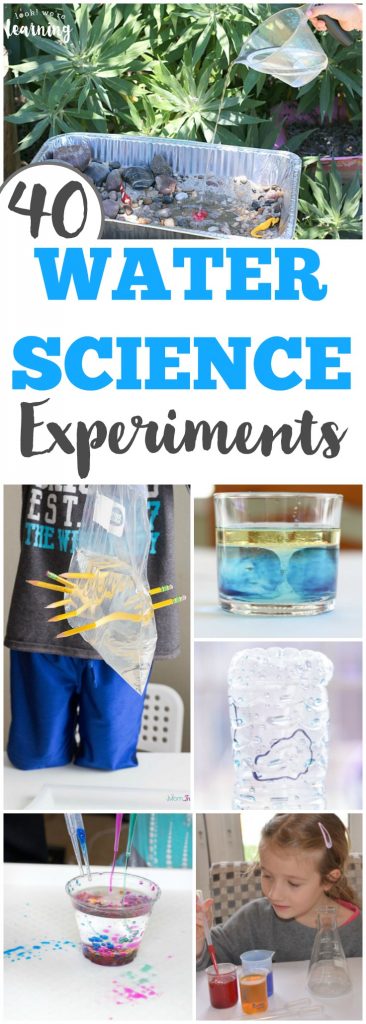
Warmer weather means lots of water play and, for us, that means plenty of simple water science experiments to try with the kids!
Since we’ve been homeschooling, I’ve learned that science doesn’t have to be a complicated subject to teach.
Many scientific concepts sound complicated (and really are amazingly intricate), but showing kids how they work doesn’t have to be complex.
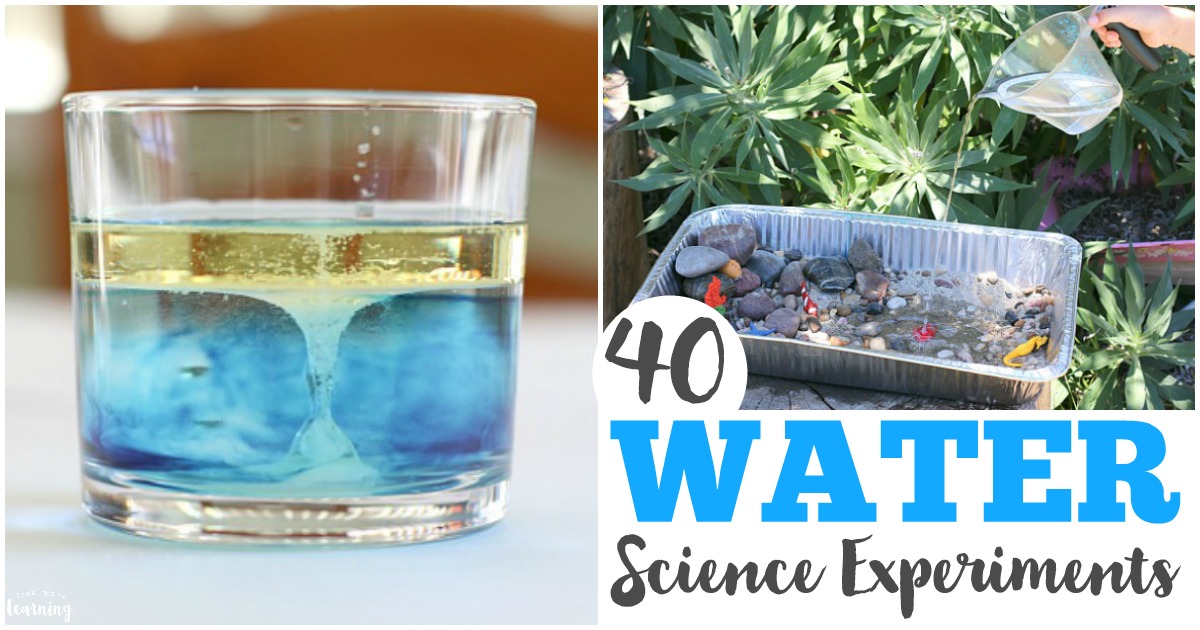
Turns out that you can use a few household supplies and demonstrate how scientific principles work in a kid-friendly way!
And that’s why I’ve rounded up 40 easy water science experiments that would be great to share with kids – either indoors or outdoors!
Don’t miss our list of science experiments that you can do in just 15 minutes too!

1. Simple Light In Water Refraction Experiment – Look! We’re Learning!
2. Milk Jug Water Wheel Experiment – J Daniel 4’s Mom
3. Float Vs Sink Experiment – Teach Me Mommy
4. Leak-Proof Bag Science Experiment – Fun Learning for Kids
5. How to Make a Lava Lamp – Coffee Cups and Crayons
6. Measuring Water Beads – Blue Bear Wood
7. Water Balloon Parachute – Fantastic Fun and Learning
8. Food Coloring Fluid Mechanics Experiment – Mama Smiles
9. Measuring Buoyancy Experiment – KC Edventures
10. Color Mixing Experiment – Science Sparks
11. Tide Pool Science Experiment – Buggy and Buddy
12. Floating Peep Boats – Sixth Bloom
13. Traveling Water Experiment – The Wise Owl Factory
14. Simple Water Displacement Experiment – Life with Moore Babies
15. Ripple Water Experiment – J Daniel 4’s Mom
16. Why Does Water Rise STEM Experiment – STEAM Powered Family
17. Make a Rain Cloud In a Jar – Coffee Cups and Crayons
18. Water Xylophone Sound Experiment – Little Bins for Little Hands
19. Growing Mint in Water – Sloely
20. Sticky Ice Experiment – Capri Plus 3

See more of these awesome simple water science experiments on page 2!
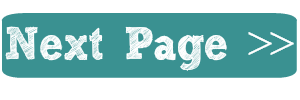
Leave a Reply Cancel reply
Your email address will not be published. Required fields are marked *
Save my name, email, and website in this browser for the next time I comment.
Stack Exchange Network
Stack Exchange network consists of 183 Q&A communities including Stack Overflow , the largest, most trusted online community for developers to learn, share their knowledge, and build their careers.
Q&A for work
Connect and share knowledge within a single location that is structured and easy to search.
Double slit experiment in water: A simple demonstration of refractive wavelength?
The speed and wavelength of light both reduce in media with high refractive index.
However, it is perhaps not intuitively clear how frequency and wavelength behave in refractive media.
A very simple demonstration would be the double slit experiment immersed or embedded in the medium. The fringe spacing could then be directly compared alongside an identical apparatus in air.
Unfortunately, I can't find references to such simple experiments.
Can anyone help?
- double-slit-experiment
- diffraction
- $\begingroup$ Rayleigh refractometer, water between lens and optical flat in Newton’s ring set up, thickness of mica sheet using double slit etc, etc, . . $\endgroup$ – Farcher Commented Apr 1, 2020 at 13:54
- $\begingroup$ Thanks. Could you provide a reference? With Newton's rings two media not one is implicitly required and the equations are complicated than for double slit. $\endgroup$ – cumfy Commented Apr 1, 2020 at 14:36
- $\begingroup$ More complicated $\endgroup$ – cumfy Commented Apr 1, 2020 at 14:37
Your intuition is correct. Same could be done in various other liquids like sugar water, mineral oil, etc.
A possible complication would be the necessity of measuring the fringe spacing in the liquid, but that can be done.
- $\begingroup$ Many thanks. Do you possibly have any references to practical demonstrations ? $\endgroup$ – cumfy Commented Apr 1, 2020 at 13:56
- $\begingroup$ Why should any one do such an experiment, you can calculate the outcome. But why not do it yourself, instead of double slit use a cd disk and one of the small laser pointers do it in air and then in water $\endgroup$ – trula Commented Aug 27, 2023 at 13:20
Your Answer
Sign up or log in, post as a guest.
Required, but never shown
By clicking “Post Your Answer”, you agree to our terms of service and acknowledge you have read our privacy policy .
Not the answer you're looking for? Browse other questions tagged waves refraction double-slit-experiment diffraction or ask your own question .
- Featured on Meta
- Preventing unauthorized automated access to the network
- User activation: Learnings and opportunities
- Join Stack Overflow’s CEO and me for the first Stack IRL Community Event in...
Hot Network Questions
- How can I make a 2D FTL-lane map on a galaxy-wide scale?
- Why is my Lenovo ThinkPad running Ubuntu using the e1000e Ethernet driver?
- Can I use named pipes to achieve temporal uncoupling?
- If Voyager is still an active NASA spacecraft, does it have a flight director? Is that a part time job?
- I currently hold an L-1 Visa and have booked to go on holiday to the US in December. Do I need an ESTA?
- Anime or manga with a tattooed character who can use a floating arrow
- All combinations of ascending/descending digits
- What is the average result of rolling Xd6 twice and taking the higher of the two sums?
- World's smallest Sudoku!
- Why did it take so long for Jitney to appear on Broadway?
- Does this work for page turns in a busy violin part?
- Is this a balanced way to implement the "sparks" spell from Skyrim into D&D?
- How can fideism's pursuit of truth through faith be considered a sound epistemology when mutually exclusive religious beliefs are accepted on faith?
- Can I find from which directory a command was executed?
- Why was Z moved to the end of the alphabet when Zeta was near the beginning?
- Shock absorber rusted from being outside
- Where is this NPC's voice coming from?
- What are major reasons why Republicans support the death penalty?
- How would humans interact with a super intelligence?
- How many natural operations on subsets are there?
- How do you tell someone to offer something to everyone in a room by taking it physically to everyone in the room so everyone can have it?
- What is an "axiomatic definition"? Every definition is an axiomatic definition?
- Matter made of neutral charges does not radiate?
- is it okay to mock a database when writing unit test?
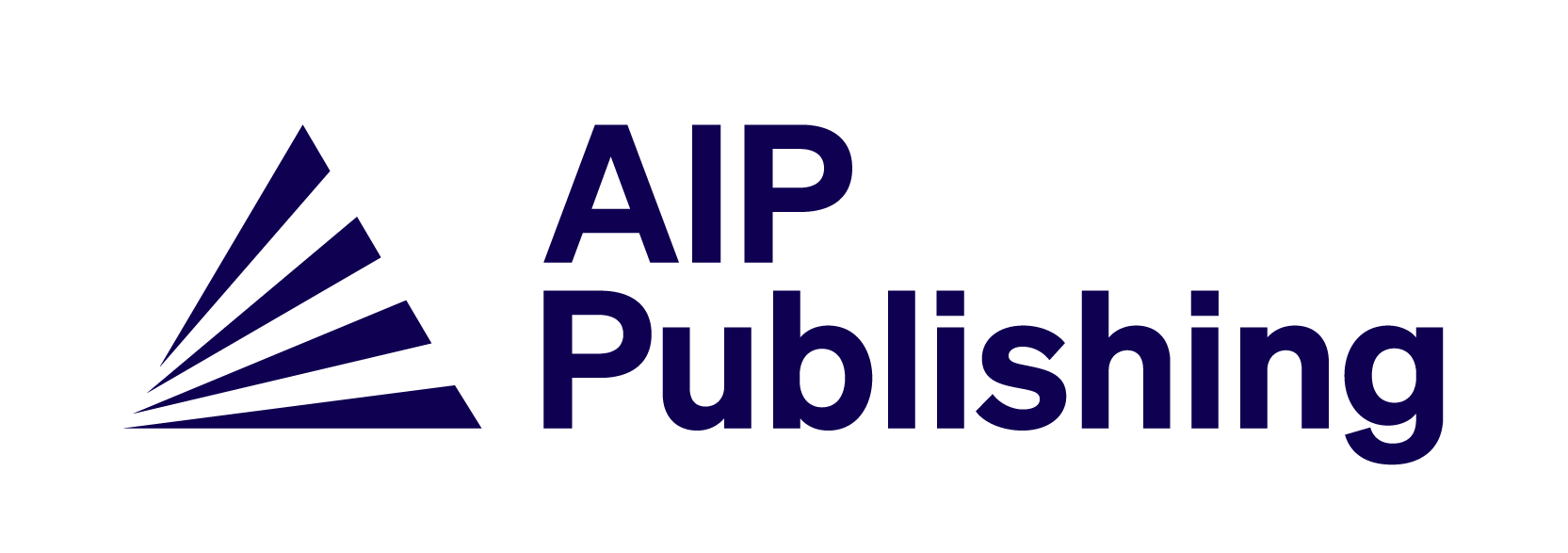
- Previous Article
- Next Article
I. INTRODUCTION
Ii. methods, iii. results and discussion, a. different 2d images to be optically transmitted, b. different objects to be reconstructed, c. different sampling ratios, iv. conclusions, supplementary material, acknowledgments, author declarations, conflict of interest, author contributions, data availability, dual-modality ghost diffraction in a complex disordered environment using untrained neural networks.

- Split-Screen
- Article contents
- Figures & tables
- Supplementary Data
- Peer Review
- Open the PDF for in another window
- Reprints and Permissions
- Cite Icon Cite
- Search Site
Yang Peng , Wen Chen; Dual-modality ghost diffraction in a complex disordered environment using untrained neural networks. APL Mach. Learn. 1 September 2024; 2 (3): 036114. https://doi.org/10.1063/5.0222851
Download citation file:
- Ris (Zotero)
- Reference Manager
We report a dual-modality ghost diffraction (GD) system to simultaneously enable high-fidelity data transmission and high-resolution object reconstruction through complex disordered media using an untrained neural network (UNN) with only one set of realizations. The pixels of a 2D image to be transmitted are sequentially encoded into a series of random amplitude-only patterns using a UNN without labels and datasets. The series of random patterns generated is sequentially displayed to interact with an object placed in a designed optical system through complex disordered media. The realizations recorded at the receiving end are used to retrieve the transmitted data and reconstruct the object at the same time. The experimental results demonstrate that the proposed dual-modality GD system can robustly enable high-fidelity data transmission and high-resolution object reconstruction in a complex disordered environment. This could be a promising step toward the development of AI-driven compact optical systems with multiple modalities through complex disordered media.
Optical modulation through disordered media has become an active research topic 1–5 and has various applications in biomedical and astronomy fields. 6,7 The main challenge is that the disordered media are inhomogeneous and variable and corrupt effective information in the wave propagation path. Some approaches to addressing this challenge have been developed, 1,4,8–10 e.g., phase conjugation, 8 memory effect, 9 and shower-curtain effect. 10 In previous studies, pixelated arrays were usually used for intensity detection, but it could be difficult (or even not available) to implement in some applications, e.g., non-visible bandwidths or low light levels.
Recently, ghost diffraction (GD) with structured illumination and a single-pixel detector 11–19 has emerged as an easy-to-implement approach. The GD was initially realized with entangled photons generated by spontaneous parametric downconversion in quantum domain. 11,12 Subsequently, experiments of GD with pseudo-thermal light were realized, which promoted its development in classical domain. 13,14 Optical information can be retrieved based on the second-order correlation between a series of illumination patterns and the realizations collected by using a single-pixel detector. 15 Advanced algorithms, e.g., differential, 20 normalized, 21 and compressed sensing, 22,23 have been developed to enhance the signal-to-noise ratio of ghost images. Furthermore, deep learning 24–26 has been applied at low samplings and could perform properly. However, training-based deep learning requires a large dataset for optimization and could lack the generalization capability. To get rid of dataset constraints, an untrained neural network (UNN) 27 was introduced 28,29 and the GD could have a comparable performance by incorporating a physical model into neural networks. Although the GD is promising in real-world scenarios 30–36 (e.g., microscopy 33 and communication 34 ), the current studies focus on developing one modality, and it is difficult to integrate dual or multiple modalities into one optical system, especially in complex disordered environments. It is desirable to explore an integrated GD system to enable multiple modalities in complex disordered environments.
Here, we report a dual-modality GD system to simultaneously enable high-fidelity optical data transmission and high-resolution ghost reconstruction through complex disordered media. The UNN is first designed to sequentially encode the pixels of a 2D image (to be optically transmitted) into a series of random amplitude-only patterns, and the zero-frequency component of the spectrum is designed to be proportional to each pixel of the 2D image. The series of generated random patterns is sequentially embedded into a spatial light modulator (SLM) in a designed optical system. Optical wave modulated by the generated random patterns illuminates an object, and a single-pixel detector is used to record a series of light intensities. High-fidelity optical data retrieval can be directly realized by using the realizations, and a high-resolution object is also recovered, which is enhanced by using block-matching and 3D filtering (BM3D) and UNN, regularized by an explicit denoiser (UNN-RED). A series of optical experiments are conducted in complex disordered environments, and the experimental results verify effectiveness and robustness of the designed dual-modality GD system.
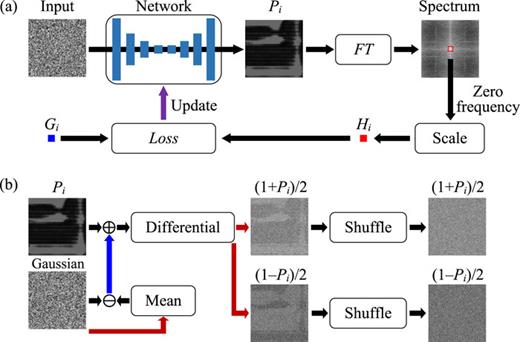
(a) Generation of random amplitude-only patterns using a UNN, and (b) Gaussian distribution and a differential approach. A zero-mean Gaussian image is obtained by subtracting its mean from a Gaussian map.
After the optimization, the zero-frequency component of the Fourier spectrum of pattern P i can be proportional to the pixel G i . The above-mentioned process is repeated until all pixels of the 2D image are encoded into random amplitude-only patterns.
Since the probability distributions of random patterns generated by the UNN are inconsistent with the light source, 38 the transmission quality could be significantly affected. Furthermore, noise could be inevitably induced by complex disordered media, preventing a direct application of the generated patterns. To overcome this challenge, a strategy is further designed, as shown in Fig. 1(b) . To align probability distributions of the generated patterns with Gaussian, a zero-mean Gaussian image is used to be superimposed with each generated pattern. Therefore, probability distribution of the pattern can be modified without affecting its original zero-frequency component in a Fourier domain. A differential approach is also employed to suppress noise. Each pattern is further divided, i.e., (1 + P )/2 and (1 − P )/2. Finally, a shuffle operation is applied to produce randomized illumination patterns.
A schematic experimental setup for the proposed dual-modality GD system is shown in Fig. 2(a) . A green laser (MGI-III-532-50 mW) with a wavelength of 532.0 nm and a peak output power of 50.0 mW is used. The laser beam is expanded by using an objective lens with a 40× magnification and then collimated. The collimated beam is reflected by a mirror and then illuminates the generated patterns embedded into SLM (Holoeye, LC-R720) with a pixel pitch of 20.0 μ m. A 4 f system is designed to project the patterns onto an object, e.g., USAF 1951 resolution target. The lenses L1 and L2 in the 4 f system have a focal length of 50.0 mm. A water tank with a dimension of 100.0 mm (length), 200.0 mm (width), and 300.0 mm (height) is placed in the optical path and is filled with 4000 ml of clean water. To create a dynamic disordered environment, 15 ml skimmed milk diluted with 1000 ml clean water is kept dropping into water tank. A rotator is used to keep operating at 600.0 revolutions per minute (rpm) to create dynamic scattering. Only one set of realizations is recorded by using a single-pixel silicon photodiode (Thorlabs, PDA100A2).
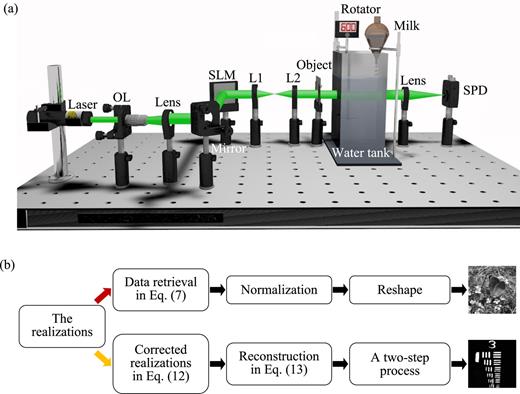
(a) Schematic experimental setup for the proposed dual-modality GD system through complex disordered media. OL: objective lens; SPD: single-pixel detector; L1 and L2: lenses. (b) A schematic of the proposed dual-modality GD system. With one set of collected realizations, the transmitted data can be retrieved in Eq. (7) , and the corrected realizations for imaging can be obtained in Eq. (12) . For data transmission, a normalization operation is further performed, and a series of the normalized data are reshaped into a 2D image. For optical imaging, a coarse image is reconstructed in Eq. (13) , followed by a two-step process.
To verify the developed dual-modality GD system, different 2D grayscale images are optically transmitted in Fig. 2(a) and are individually encoded into a series of random amplitude-only patterns using the designed UNN. The generated patterns are sequentially displayed by SLM to illuminate an object (i.e., USAF 1951 resolution target) through disordered media. Figure 3 shows the experimental results in the proposed dual-modality GD system through static and dynamic disordered media, respectively. In static disordered environments, the experimentally retrieved data are shown in Figs. 3(a) – 3(d) , and the reconstructed ghost images are shown in Figs. 3(e) – 3(h) . Quality of the experimentally retrieved images is quantitatively evaluated by using peak signal-to-noise ratio (PSNR) and structural similarity index measure (SSIM). 46 As shown in Figs. 3(a) – 3(d) , the retrieved data have high PSNR and high SSIM, and it is demonstrated that the proposed dual-modality GD system enables high-fidelity image transmission.
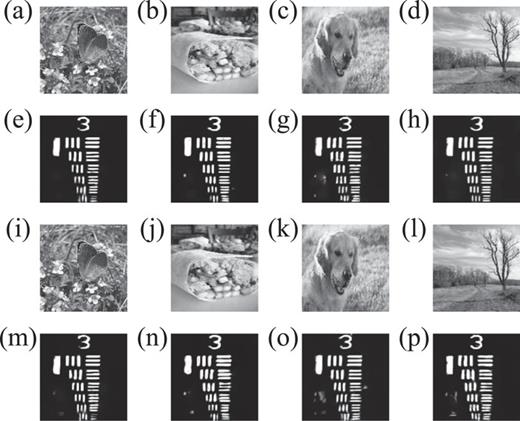
Experimental results: (a)–(d) the retrieved 2D images in static disordered media. PSNR values are 39.27, 40.25, 39.12, and 38.24 dB, and SSIM values are 0.9975, 0.9884, 0.9867, and 0.9884; (e)–(h) the reconstructed objects in static disordered media with CNR of 24.22, 24.83, 28.16, and 25.02, respectively; (i)–(l) the retrieved 2D images in dynamic disordered media. PSNR values are 39.71, 38.62, 39.09, and 40.16 dB, and SSIM values are 0.9973, 0.9810, 0.9899, and 0.9877; (m)–(p) the reconstructed objects in dynamic disordered media with CNR of 23.53, 21.09, 23.68, and 20.67, respectively. The size of the reconstructed object images is 128 × 128 pixels. For CNR calculations, signal part and background part are shown in Fig. S1 (see the supplementary material ).
To illustrate the quality of the retrieved images, the pixels along the 30th row of the retrieved images shown in Figs. 3(a) and 3(b) are shown in Figs. 4(a) and 4(b) , respectively. It is demonstrated that the experimentally retrieved data overlap with the original data. PSNR values of Figs. 4(a) and 4(b) are 39.75 and 39.39 dB, respectively. MSE values are 1.06 × 10 −4 and 1.15 × 10 −4 , respectively. The high PSNR and low MSE demonstrate that the proposed optical system is feasible and robust for optically transmitting 2D grayscale images. Contrast-to-noise ratio (CNR) 47–49 is calculated to evaluate quality of the reconstructed ghost images. In Figs. 3(e) – 3(h) , the reconstructed ghost images have high CNR. It is demonstrated that the proposed dual-modality GD system can reconstruct a high-quality object at the same time. Here, element 5 in Group 3 is the finest resolvable feature, and high spatial resolution of 78.74 μ m is achieved. The experimental results shown in Figs. 3(a) – 3(h) demonstrate that the proposed dual-modality GD system can simultaneously realize high-fidelity optical data transmission and high-resolution object reconstruction in static disordered environments.
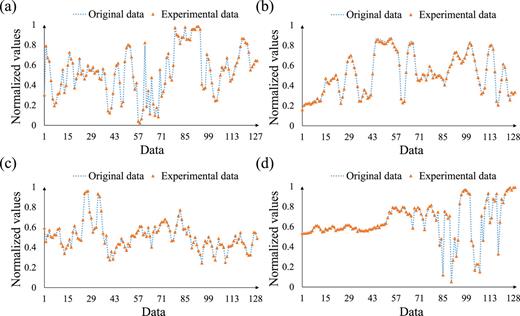
(a)–(d) Comparisons between the pixels along the 30th row of the experimentally retrieved images shown in Figs. 3(a) , 3(b) , 3(k) , and 3(l) and those of original images. Original data refer to a row of the 2D grayscale image to be transmitted and are encoded into a series of random patterns via the UNN.
When optical experiments in dynamic disordered environments are conducted, optical transmission and imaging results are shown in Figs. 3(i) – 3(p) . In Figs. 3(i) – 3(l) , the experimentally retrieved 2D images are of high fidelity. Typical comparisons using the retrieved data at the 30th row of Figs. 3(k) and 3(l) are shown in Figs. 4(c) and 4(d) . PSNR of the data in Figs. 4(c) and 4(d) is 40.87 and 40.31 dB, respectively. MSE values are 8.19 × 10 −5 and 9.31 × 10 −5 , respectively. It is experimentally demonstrated that the retrieved data are in accordance with the original data. The reconstructed ghost images are shown in Figs. 3(m) – 3(p) , and element 5 in Group 3 is well-resolved. It is demonstrated that high spatial resolution of 78.74 μ m is achieved in the proposed dual-modality GD system through dynamic disordered media. Dual modalities, i.e., high-fidelity optical data transmission and high-resolution object reconstruction, are realized in the proposed optical system through complex disordered media.
A 2D image (“butterfly”) is encoded into a series of random amplitude-only patterns, which are sequentially used to illuminate an object, and different objects (i.e., “1X,” “95,” “AF,” and “Triple-bar”) are individually tested in the optical path. Static (clean) water and dynamic (turbid) water are applied in Fig. 2(a) . In the optical setup with static water, the experimental results are shown in Figs. 5(a) – 5(h) . It is shown in Figs. 5(a) – 5(d) that the retrieved images are of high fidelity. PSNR values are higher than 40.0 dB, and SSIM values are close to 1. The comparisons in the 30th row of Figs. 5(a) and 5(b) are shown in Figs. 6(a) and 6(b) . PSNR values are 41.00 and 43.45 dB, respectively. MSE values are 7.94 × 10 −5 and 4.52 × 10 −5 , respectively. It is experimentally demonstrated that high-fidelity data transmission can always be realized, when different objects are placed in Fig. 2(a) . The reconstructed objects are shown in Figs. 5(e) – 5(h) . It can be seen that the recovered objects are of high quality with CNR values higher than 33.0.
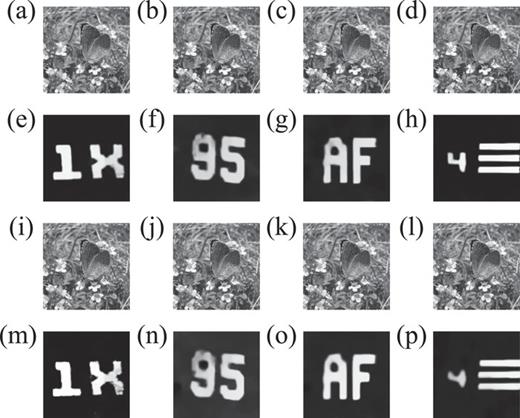
Experimental results: (a)–(d) the retrieved 2D transmitted images in static disordered media. PSNR values are 41.51, 41.49, 41.21, and 40.19 dB, and SSIM values are 0.9979, 0.9982, 0.9980, and 0.9972; (e)–(h) the reconstructed objects in static disordered media with CNR of 33.22, 40.11, 36.26 and 39.71, respectively; (i)–(l) the retrieved 2D transmitted images in dynamic disordered media. PSNR values are 39.96, 39.91, 38.12, and 38.51 dB, and SSIM values are 0.9976, 0.9978, 0.9970, and 0.9969; (m)–(p) the reconstructed objects in dynamic disordered media with CNR of 30.66, 22.19, 25.45 and 25.82, respectively. For CNR calculations, the signal part and background part are shown in Fig. S1 (see the supplementary material ).
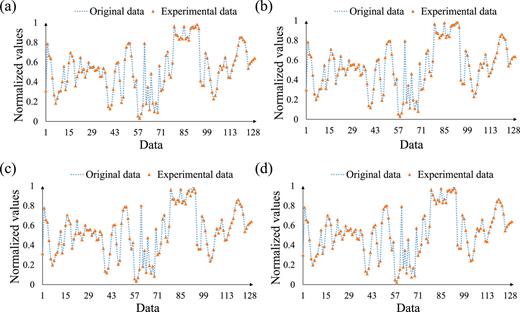
(a)–(d) Comparisons between the pixels along the 30th row of the experimentally retrieved images shown in Figs. 5(a) , 5(b) , 5(k) , and 5(l) and those of the original image.
In dynamic disordered environments, the experimental results are shown in Figs. 5(i) – 5(p) . In Figs. 5(i) – 5(l) , high PSNR and high SSIM are achieved. The pixels along the 30th row of the retrieved 2D images in Fig. 5(k) and 5(l) are shown in Figs. 6(c) and 6(d) , respectively. PSNR values of the experimentally retrieved data in Figs. 6(c) and 6(d) are 38.70 and 40.23 dB, respectively. MSE values are 1.35 × 10 −4 and 9.49 × 10 −5 , respectively. It is demonstrated that the retrieved data are of high fidelity in dynamic disordered environments. The reconstructed objects are shown in Figs. 5(m) – 5(p) . The reconstructed images render detailed object information with high visibility. It is experimentally verified that the proposed dual-modality GD system has high robustness to simultaneously reconstruct a high-quality object and retrieve high-fidelity data using only one set of realizations in dynamic and complex disordered media.
The proposed optical system is further verified through disordered media at different sampling ratios. Imaging through static (clean) water and dynamic (turbid) water is conducted, and the experimental results are shown in Fig. 7 when sampling ratios of 12.2%, 24.4%, 36.6%, 48.8%, 61.0%, 73.2%, 85.4%, and 97.6% are used, respectively. The comparisons in Fig. 7 show effectiveness of the proposed two-step enhancement approach. It is shown in Figs. 7(a) and 7(b) that the reconstruction quality is dramatically enhanced with the higher sampling ratio. With the developed two-step enhancement, object images with the higher visibility can always be obtained. When the sampling ratio is not smaller than 24.4%, the reconstructed ghost images can contain clear information. When dynamic and turbid water is considered, the experimental results are shown in Figs. 7(c) and 7(d) . With the developed two-step enhancement, the visibility is significantly enhanced and noise is highly suppressed, as shown in Fig. 7(d) . It is observed that the proposed optical system can recover high-quality objects at low sampling rates (e.g., 24.4%) in dynamic disordered environments. In the proposed dual-modality GD system, the sampling ratio is constrained by the length of the transmitted data. The experimental results in Fig. 7 demonstrate that high-quality objects can still be reconstructed in complex disordered environments even when the length of the transmitted data is small.
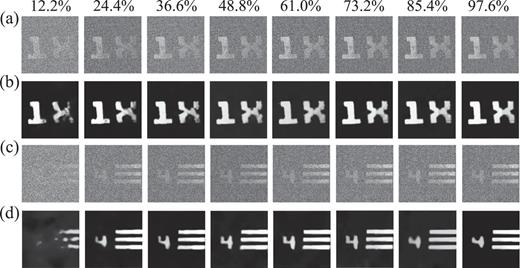
Experimental results at different sampling ratios: (a) without the two-step enhancement in static environments, (b) with the two-step enhancement in static environments, (c) without the two-step enhancement in dynamic environments, and (d) with the two-step enhancement in dynamic environments.
In Figs. 8(a) – 8(d) , CNR is calculated to quantitatively illustrate the quality of the reconstructed objects at different sampling ratios. In Figs. 8(a) and 8(b) , CNR values of the reconstructed object images have similar variation trends, steadily increasing with the higher sampling ratio. CNR values of the reconstructed objects with BM3D and UNN-RED, as shown in Fig. 8(b) , are much higher than those without enhancement as shown in Fig. 8(a) . When optical experiments in dynamic turbid water are conducted, the trends of CNR variations are similar, as shown in Figs. 8(c) and 8(d) . The average CNR values of the reconstructed object images without enhancement are in a range of 0.34–1.56, and the average CNR values with the two-step enhancement increase from 5.27 to 24.17.
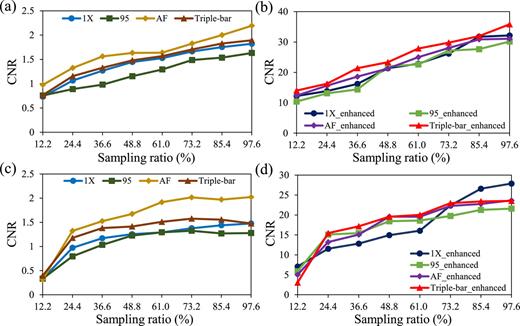
Variation of CNR values at different sampling ratios: (a) without the two-step enhancement in static disordered environments, (b) with the two-step enhancement in static disordered environments, (c) without the two-step enhancement in dynamic disordered environments, and (d) with the two-step enhancement in dynamic disordered environments.
We have reported a dual-modality GD system using a UNN, simultaneously enabling high-fidelity data transmission and high-resolution object reconstruction through complex disordered media using only one set of realizations. A series of random amplitude-only patterns are generated to carry information of a 2D grayscale image (to be optically transmitted) using a UNN. The generated random patterns are embedded into SLM to modulate optical wave, and a series of single-pixel light intensities are recorded at the receiving end. With only one set of realizations, high-fidelity data information can be retrieved, and a high-resolution and high-visibility object can be recovered. A series of optical experiments have been conducted to verify the proposed dual-modality GD system. It is demonstrated that a dual-modality GD system can be realized in a complex disordered environment. The proposed approach could open an avenue for the development of AI-driven multi-modality optical systems in complex disordered environments.
Additional information supporting the findings of this work is provided as a separate file. The supplementary material includes information about the contrast-to-noise ratio (CNR).
This work was supported by the Hong Kong Research Grants Council (Grant Nos. 15224921, 15223522), the Basic and Applied Basic Research Foundation of GuangDong Province (Grant No. 2022A1515011858), and the Hong Kong Polytechnic University (Grant No. 1-WZ4M).
The authors have no conflicts to disclose.
Yang Peng : Data curation (equal); Formal analysis (equal); Investigation (equal); Methodology (equal); Software (equal); Visualization (equal); Writing – original draft (equal); Writing – review & editing (equal). Wen Chen : Conceptualization (lead); Formal analysis (equal); Funding acquisition (lead); Investigation (equal); Methodology (equal); Project administration (lead); Resources (equal); Supervision (lead); Writing – review & editing (equal).
The data and source codes that support the findings of this study are openly available in GitHub at https://github.com/YangPeng2021/Dual-modality-ghost-diffraction .
Citing articles via
Related content.
- Online ISSN 2770-9019
- For Researchers
- For Librarians
- For Advertisers
- Our Publishing Partners
- Physics Today
- Conference Proceedings
- Special Topics
pubs.aip.org
- Privacy Policy
- Terms of Use
Connect with AIP Publishing
This feature is available to subscribers only.
Sign In or Create an Account

IMAGES
VIDEO
COMMENTS
Experiment with diffraction through elliptical, rectangular, or irregular apertures. Make waves with a dripping faucet, audio speaker, or laser! Add a second source to create an interference pattern. Put up a barrier to explore single-slit diffraction and double-slit interference. Experiment with diffraction through elliptical, rectangular, or ...
where λ λ is the wavelength in vacuum and n is the medium's index of refraction. It follows that the wavelength of light is smaller in any medium than it is in vacuum. In water, for example, which has n = 1.333, the range of visible wavelengths is (380 nm)/1.333 to (760 nm)/1.333, or λ n = λ n = 285-570 nm. Although wavelengths change while traveling from one medium to another, colors ...
Diffraction experiments are best done before interference experiments. They show how a straight parallel wave can become a point source producing a circular wave at the gap in a barrier. ... First set up the ripple tank with about 1 cm depth of water. Set up a line of small barriers 5 cm from the vibrator, as shown. There should be a gap of 2 ...
The narrower the slit, the more the light spreads out. In fact, the angle between two adjacent dark bands in the diffraction pattern is inversely proportional to the width of the slit. Thin objects, such as a strand of hair, also diffract light. Light that passes around the hair spreads out, overlaps, and produces a diffraction pattern.
A ripple tank is placed above a mirror and a projection screen. A horizontal bar, whose frequency can be varied, taps the surface of the water and produces p...
Diffraction is the interference or bending of waves around the corners of an obstacle or through an aperture into the region of geometrical shadow of the obstacle/aperture. ... Thomas Young's sketch of two-slit diffraction for water waves, ... In the case of Young's double-slit experiment, this would mean that if the transverse coherence length ...
14.2 Young's Double-Slit Experiment In 1801 Thomas Young carried out an experiment in which the wave nature of light was demonstrated. The schematic diagram of the double-slit experiment is shown in Figure 14.2.1. Figure 14.2.1 Young's double-slit experiment. A monochromatic light source is incident on the first screen which contains a slit .
Light is so common that we rarely think about what it really is. But just over two hundred years ago, a groundbreaking experiment answered the question that ...
In order to study the diffraction pattern on a screen, the single-slit experiment is employed. Consider a monochromatic source of light that passes through a slit AB of width a as shown in the figure. At point P on the screen, the secondary waves interfere destructively and produce a dark fringe.
Edexcel. Religious Studies. Past Papers. OCR. Religious Studies. Revision notes on 4.11.4 Electron Diffraction for the OCR A Level Physics syllabus, written by the Physics experts at Save My Exams.
Diffraction is often demonstrated with water waves in a ripple tank. A set of straight (or 'plane') waves passes through a gap in a barrier. Curved waves come out the other side: The effect is greatest when the wavelength of the waves is similar in size to the gap or object size. Notice that the wavelength is the same on both sides - spreading ...
Generate plane water waves and use a barrier to demonstrate diffraction at an edge. Then, form a slit and observe diffraction behind the slit. Repeat this experiment for a double-slit. By using the integrated wave generator as well as the external wave generator, generate two circular waves and observe the interference.
Explore wave interference with interactive simulations, including single-slit diffraction and double-slit interference patterns.
Diffraction is defined as the bending of waves around the corners or opening of an obstacle, and it can happen to any forms of waves, including water waves, light waves, and sound waves. Diffraction can be demonstrated by placing an obstacle in a ripple tank and observing the water wave's path. You will notice that the waves that pass the ...
Water surface waves travel along the boundary between air and water. The restoring forces of the wave motion are surface tension and gravity. At different water depths, these two forces play different roles. The ripple tank can be used to study almost all the wave properties: reflection, refraction, interference and diffraction.
This experiment demonstrates diffraction using water waves in a ripple tank. You can also demonstrate diffraction using a single slit and a light source with coloured filters. ... Diffraction. Water waves in a ripple tank can be used to demonstrate diffraction and interference. Turn on the wave generator so that it produces waves with a high ...
Consider figure 2, which shows the set-up for a diffraction grating experiment. If a monochromatic light source shines on the grating, images of the light will appear at a number of angles—θ 1, θ 2, θ 3 and so on. The value of θ m is given by the grating equation shown above, so that θ m = arcsin mλ d
Diffraction and Interference. We know that visible light is the type of electromagnetic wave to which our eyes responds. As we have seen previously, light obeys the equation. c = fλ, where c = 3.00 × 108 m/s is the speed of light in vacuum, f is the frequency of the electromagnetic wave in Hz (or s -1), and λ is its wavelength in m.
3.2 Setting up the DataStudio software. Log in to the computer and double-click the Physics Experiment Resources folder, then Interference and Diffraction of Light and DataStudio Files. Double-click the file Diffraction.ds to open the DataStudio activity. Check the Experiment Setup window.
Experiment 9: Interference and Diffraction Answer these questions on a separate sheet of paper and turn them in before the lab 1. Measuring the Wavelength of Laser Light In the first part of this experiment you will shine a red laser through a pair of narrow slits (a = 40 µm) separated by a known distance (you will use both d = 250 µm and 500 ...
Water Refraction Science Experiment. What you need: A paper with a design on it. If you wish, you can download and print our printable. It is two pages and includes the colored bars and two arrows. Instructions: Place a jar or glass about 6 inches in front of the colored bars or arrows. Pour in the water.
17. Make a Rain Cloud In a Jar - Coffee Cups and Crayons. 18. Water Xylophone Sound Experiment - Little Bins for Little Hands. 19. Growing Mint in Water - Sloely. 20. Sticky Ice Experiment - Capri Plus 3. See more of these awesome simple water science experiments on page 2!
A very simple demonstration would be the double slit experiment immersed or embedded in the medium. The fringe spacing could then be directly compared alongside an identical apparatus in air. Unfortunately, I can't find references to such simple experiments.
Recently, ghost diffraction (GD) with structured illumination and a single-pixel detector 11-19 has emerged as an easy-to-implement approach. The GD was initially realized with entangled photons generated by spontaneous parametric downconversion in quantum domain. 11,12 Subsequently, experiments of GD with pseudo-thermal light were realized, which promoted its development in classical domain ...