The Science Notes
Your Best Science Education Portal

X-ray Crystallography: Definition, Principle, Steps, Data Analysis, Applications, and Limitations
What is x-ray crystallography.
X-ray crystallography is a scientific technique used to determine the arrangement of atoms within a crystal. It involves exposing a crystal to X-rays and analyzing the resulting diffraction patterns to deduce the three-dimensional arrangement of atoms in the crystal lattice.
Principle of X-ray Crystallography
The principle of X-ray crystallography is based on how X-rays interact with the electrons within a crystal lattice. When X-rays pass through a crystal, they engage with the atoms’ electrons, leading to an important phenomenon called diffraction. Diffraction happens when waves encounter periodic structures, causing them to scatter in specific directions. In X-ray crystallography, the crystal lattice serves as such a periodic structure, causing the X-rays to undergo diffraction.
The resulting diffraction pattern carries valuable information about the arrangement of atoms within the crystal. The positions and distances between atoms influence the angles and intensities of the diffracted X-rays. By precisely measuring these angles and intensities, researchers can extract essential details about the crystal’s structure.
The principle of X-ray crystallography relies on the assumption that the crystal has a well-ordered arrangement, with atoms repeating in a pattern. Therefore, acquiring high-quality crystals is vital for successful X-ray crystallography experiments.
Instrumentation
The instrumentation used in X-ray crystallography plays a critical role in the process of determining the atomic structure of crystals. The main instruments involved include:
- X-ray Source: X-ray crystallography requires a high-intensity X-ray source, typically an X-ray generator or a synchrotron radiation facility. These sources emit X-rays with a specific wavelength, usually in the range of 0.6 to 2.5 angstroms (Å), which is appropriate for interacting with the crystal lattice.
- Monochromator: To obtain a monochromatic X-ray beam with a specific wavelength, a monochromator is used. It filters out unwanted X-ray wavelengths, allowing only the desired wavelength to pass through. Monochromators can be made of various materials, such as graphite or crystals like quartz or silicon.
- Collimator: The X-ray beam emitted from the source needs to be collimated to ensure a well-defined and focused beam. Collimators are used to narrow down the X-ray beam, reducing its divergence and scattering before it reaches the crystal sample.
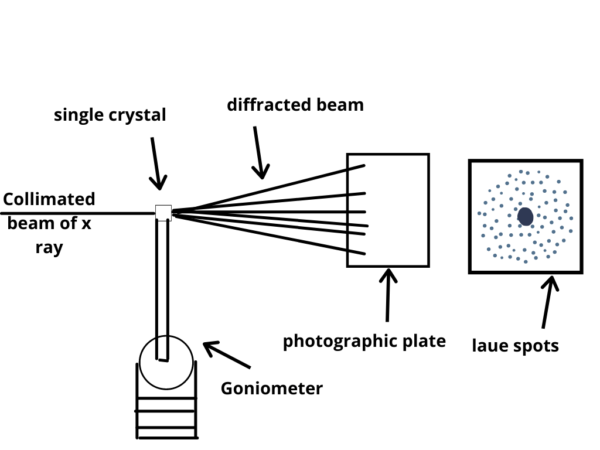
- Sample Mounting System: The crystal sample needs to be securely mounted and positioned for X-ray analysis. Sample mounting systems, such as goniometers, allow precise rotation and translation of the crystal in order to collect diffraction data from different crystallographic orientations.
- X-ray Detector: X-ray detectors capture the diffraction pattern produced when X-rays interact with the crystal lattice. There are several types of detectors used in X-ray crystallography, including photographic films, image plates, gas detectors, and more commonly, area detectors such as CCD (charge-coupled device) or CMOS (complementary metal-oxide-semiconductor) detectors. These detectors record the intensities and positions of the diffracted X-rays.
- Cryocooling System: Many crystallographic studies require samples to be cooled to very low temperatures, typically around -173 degrees Celsius or lower, to reduce radiation damage and minimize sample motion. Cryocooling systems, such as liquid nitrogen or helium cryostats, are used to cool the crystal sample during data collection.
- Data Acquisition System: X-ray crystallography generates a vast amount of diffraction data that needs to be efficiently captured and processed. Data acquisition systems, often integrated with the X-ray detectors, enable the collection and storage of diffraction images.
- Computational Tools: Once the diffraction data is obtained, powerful computational tools and software packages are used for data analysis, structure determination, and refinement. These tools employ mathematical algorithms and techniques, such as Fourier transforms, least-squares fitting, and phase determination methods, to extract meaningful structural information from the diffraction data.
Steps of X-ray Crystallography
X-ray crystallography involves several key steps in order to determine the atomic structure of a crystal. Here are the main steps involved in the process:
Crystallization:
The first step is to obtain a high-quality crystal of the substance of interest. This can be a challenging task as it requires finding suitable conditions for the molecules to arrange themselves in an ordered crystal lattice. Crystallization methods can vary depending on the nature of the substance being studied.
Data Collection:
Once a suitable crystal is obtained, it is mounted on a goniometer and placed in an X-ray beam. The crystal is then rotated to collect a series of X-ray diffraction images. The diffraction data is typically collected as a series of two-dimensional images, known as “diffraction patterns,” which represent the scattering of X-rays by the crystal.
The diffraction patterns are analyzed to determine the orientation and symmetry of the crystal lattice. This process, known as indexing, involves identifying the systematic patterns of spots in the diffraction images and assigning them to specific crystallographic planes.
Data Reduction:
The collected diffraction data is processed and reduced to obtain the intensities of the diffracted X-rays and their corresponding angles. This step involves correcting for various experimental factors, such as detector distortions and absorption effects.
Structure Determination:
The reduced diffraction data is used to determine the electron density distribution within the crystal. This is done by solving a set of mathematical equations known as the phase problem. The phase problem refers to the challenge of determining the phase information of the diffracted X-rays, which is necessary to reconstruct the electron density map.
Model Building and Refinement:
Using the electron density map, an initial model of the crystal structure is built by placing atoms in reasonable positions. This model is refined iteratively by comparing the calculated diffraction pattern from the model with the experimental data. The refinement process adjusts the positions of the atoms and optimizes other parameters until the calculated and observed data match as closely as possible.
Validation:
The final refined model is subjected to various validation procedures to assess its quality and reliability. This includes checking for reasonable bond lengths, angles, and overall geometry. Validation also involves evaluating the fit of the model to the experimental data and assessing its statistical measures.
Structure Analysis and Interpretation:
Once the crystal structure is determined and validated, it is analyzed and interpreted to gain insights into the arrangement of atoms and their interactions within the crystal. This analysis can provide information about chemical bonding, molecular conformations, and other structural properties.
These steps collectively allow scientists to obtain a detailed understanding of the atomic arrangement and three-dimensional structure of a crystal using X-ray crystallography.
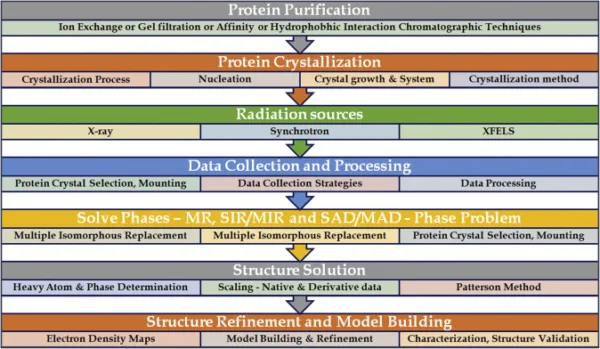
Mathematics in Data Analysis of X-ray Crystallography
The data analysis in X-ray crystallography involves several mathematical formulas and equations to extract information about the atomic structure of a crystal. Here are some key formulas used in the data analysis process:
1. Bragg’s Law:
Bragg’s Law relates the angle of diffraction (θ) of X-rays from a crystal lattice to the wavelength of the X-rays (λ) and the spacing between crystal planes (d):
nλ = 2d sin(θ)
Here, n is an integer representing the order of the diffraction peak.
2. Structure Factors:
Structure factors (F_hkl) are complex numbers that represent the amplitude and phase of the diffracted X-rays from each set of crystallographic planes. They are calculated using the formula:
F_hkl = Σ[ f_j e^(-2πi(hx_j + ky_j + lz_j)) ]
Here, f_j is the atomic scattering factor for atom j, (hkl) represents the Miller indices of the crystallographic plane, and (x_j, y_j, z_j) are the coordinates of atom j.
3. Phase Determination:
Solving the phase problem in X-ray crystallography is crucial to determine the electron density distribution. Various methods, such as direct methods or molecular replacement, involve mathematical algorithms to determine the phases of the structure factors.
4. Fourier Transform:
The electron density map of the crystal is obtained by performing a Fourier transform of the structure factors. The equation for the Fourier transform is:
ρ(r) = Σ[ F_hkl e^(2πi(hx + ky + lz)) ]
Here, ρ(r) represents the electron density at position r in the crystal lattice.
5. Rietveld Refinement:
Rietveld refinement is a common method used to refine the crystal structure model and minimize discrepancies between the calculated and observed diffraction data. It involves the use of least-squares fitting algorithms to adjust atomic positions, thermal vibrations, and other parameters.
These formulas provide a general overview of the mathematical aspects involved in X-ray crystallography data analysis. However, it’s important to note that the specific calculations and algorithms used may vary depending on the software packages and techniques employed. The field of X-ray crystallography relies heavily on computational methods and mathematical analysis to extract meaningful structural information from diffraction data.
Applications of X-ray Crystallography
- Structural Biology: Reveals protein and nucleic acid structures for drug discovery.
- Drug Discovery and Design: Optimizes drug candidates and understands binding modes.
- Material Science: Analyzes crystal structures for developing new materials.
- Chemical Crystallography: Determines molecular structures and studies reaction mechanisms.
- Geological Studies: Identifies minerals and studies geological processes.
- Pharmaceutical Industry: Characterizes drug properties for formulation stability.
- Nanotechnology: Designs nanomaterials with specific functionalities.
- Protein Engineering and Enzyme Catalysis: Enhances understanding of enzyme structures.
- Physical and Solid-State Chemistry: Investigates solid-state materials’ properties.
Limitations of X-ray Crystallography
- Requires high-quality single crystals.
- Relies on large sample sizes.
- Time-consuming process.
- Radiation damage can alter crystal structure.
- Limited to crystalline materials.
- The phase problem requires additional approaches.
- Assumes perfect order and static structure.
- Some samples may be sensitive to X-ray radiation.
Despite limitations, X-ray Crystallography remains valuable for studying atomic structures.
- Rupp, B. (2010). “An Introduction to X-ray Crystallography.” In Protein Crystallography: Methods and Protocols (2nd ed., pp. 1-38). Humana Press. DOI: 10.1007/978-1-60761-795-2_1.
- Rhodes, G. (2017). “Principles of X-ray Crystallography.” In Crystallography Made Crystal Clear: A Guide for Users of Macromolecular Models (3rd ed., pp. 1-34). Academic Press. DOI: 10.1016/B978-0-12-802950-2.00001-9.
- Cullity, B. D., & Stock, S. R. (2001). “Diffraction: The Study of Crystalline Materials through X-rays, Electrons, and Neutrons.” In Elements of X-ray Diffraction (3rd ed., pp. 1-56). Prentice Hall.
- Prince, E. (2004). “X-ray Diffraction.” In Mathematical Techniques in Crystallography and Materials Science (2nd ed., pp. 173-232). Springer. DOI: 10.1007/0-306-48555-7_5.
- Helliwell, J. R. (2012). “X-ray Crystallography: An Introduction to the Techniques.” Oxford University Press. ISBN: 978-0199659845.
Learn more:
DRUG DISCOVERY AND DEVELOPMENT: A STEP-BY-STEP PROCESS

Leave a Reply Cancel reply
Your email address will not be published. Required fields are marked *
Explainer: what is X-ray crystallography?
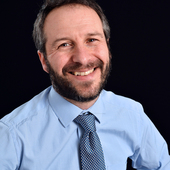
Senior Lecturer in Biological Chemistry, University of Hull
Disclosure statement
Mark Lorch does not work for, consult, own shares in or receive funding from any company or organisation that would benefit from this article, and has disclosed no relevant affiliations beyond their academic appointment.
University of Hull provides funding as a member of The Conversation UK.
View all partners
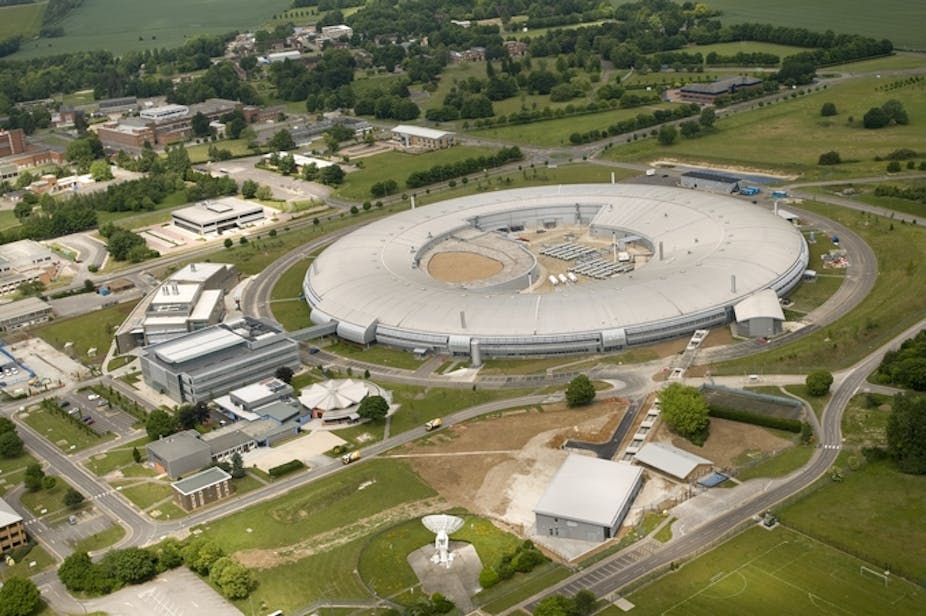
Around 100 years ago a father and his son in north England conducted an experiment that would revolutionise the way scientists study molecules. A refined version of their method still remains one of the most important tools for scientists.
To achieve this feat, William Henry Bragg and his son William Lawrence Bragg used table salt (sodium chloride). They prepared a clean crystal of salt and shone X-rays on it, which created a beautiful geometric pattern on a photographic paper placed behind it.
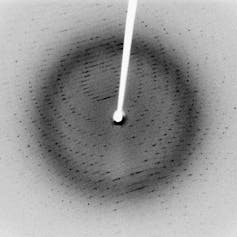
Others had done similar things before, but the Braggs made an intuitive leap. They realised that tucked away in the layout of the dots was information about salt’s molecular structure. Lawrence Bragg then came up with a formula, now known as Bragg’s law, that could be used to extracted this information allowing him to work out how the atoms of sodium and chlorine are arranged in a salt crystal.
Today we know that inside crystals there are atoms arranged in regular patterns. Each of these atoms has a dense core containing neutrons and protons, and less dense outer shells containing electrons. X-rays that hit these obstacles interact with other X-rays that cause a phenomenon called diffraction, something all waves (light or sound) undergo whenever they hit obstacles. The result of this diffraction is what was captured on the photographic plate by the Bragg team.
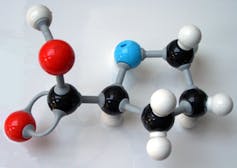
Even if you never paid attention in a chemistry lesson, you may recognise the ball-and-stick representation of chemical structures, where balls are the atoms and sticks the bonds that hold them together. But this was not known to the Braggs. Their work not only confirmed the existence of atoms but also showed how they come together to form compounds.
From that moment on, the mysterious way that atoms interact with each other was within the grasp of scientists. They could begin to unravel the structures of molecules. In the last century, 27 Nobel prizes have been awarded for discoveries directly resulting from the use X-ray crystallography. The impact of the Yorkshire-born family’s work can be put no better than by Max Perutz, a recipient of one of those 27 Nobels:
Why water boils at 100ºC and methane at -161ºC, why blood is red and grass is green, why diamond is hard and wax is soft, why glaciers flow and iron gets hard when you hammer it, how muscles contract, how sunlight makes plants grow and how living organisms have been able to evolve into ever more complex forms … the answers to all these problems have come from structural analysis.
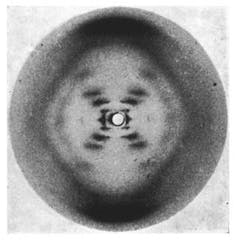
In 1953 Rosalind Franklin used X-ray crystallography to produce images from a crystal of DNA. One of those images, called photograph 51, eventually led James Watson, Francis Crick and Maurice Wilkins to describe the helical structure of DNA is dubbed the most important “photograph” ever taken.
These images taken by crystallographers are known as diffraction patterns, and now we can create such patterns using laser pointers . When the light from a laser passes through a helical spring it produces a characteristic “X” mark on the wall behind.
To most, and without an understanding of Bragg’s Law, it is difficult to make the leap from a spotty cross to underlying structure of invisible atoms. But the result bears a striking similarity to Franklin’s famous image. The angle of the cross and the distance between the spots contains information about the thickness of the wire and tightness of the spring, compressing the spring, for instance, changes the pattern produce. Similarly photo 51 contains all the data required to unlock the structure of DNA.
DNA is probably the most famous structure determined by X-ray crystallography, but it is just one among thousands: from table salt to drugs and from materials to massive intricate proteins the structure of which drives our understanding of the process that makes life possible . X-ray crystallography’s impact is one reason why the UN has called 2014 the International Year of Crystallography .
The future is bright
But chemists have a love-hate relationship with X-ray crystallography, because it still has one major limitation. As its name suggests, the method requires crystals and they need to be of certain quality and size. Growing good salt crystals is easy, but producing a crystal of a complex molecule like a protein is often met with failure. Many scientists have built their careers by just learning how to build good quality crystals of a particular protein.
That is why today’s advances in X-ray crystallography are driven, in part, by attempts to circumvent the crystal problem. One way to do this is to use more powerful X-rays. Just as a bright torch is more revealing than a candle, the more energy in an X-ray beam the smaller the crystal required to get a good diffraction pattern.
The X-ray source used by the Braggs was a small glass tube resembling a light bulb. It produced X-rays just strong enough to reveal the structure of a simple salt crystal. In contrast modern light sources are vast particle accelerators called synchrotrons, like the Diamond Light Source . These instruments can be hundreds of meters across and produce beams tens of thousands of times more powerful than the Sun itself. And with these incredible beams scientists can extract structures from smaller and smaller crystals, until it become possible to do away with crystals .
Other articles in this series: The little known science that improved everything around us
- Crystallography
- Nobel Prize

Chief People & Culture Officer
Lecturer / senior lecturer in construction and project management.

Lecturer in Strategy Innovation and Entrepreneurship (Education Focused) (Identified)

Research Fellow in Dynamic Energy and Mass Budget Modelling

Communications Director
X-Ray Crystallography, Basic Principles
- Reference work entry
- pp 2026–2029
- Cite this reference work entry
- Richard A. Engh 1
1754 Accesses
1 Citations
X-ray crystallography reveals the spatial structure of molecules by measuring how they scatter X-ray radiation when arranged in a crystal lattice . Two broad fields may be distinguished: small molecule crystallography deals with a small number of atom positions and typically well-ordered crystals, while macromolecular (usually protein) crystallography determines a much larger number of atomic positions, usually despite considerable crystalline disorder .
Their common goal is to calculate the electron density distribution in the crystal from measured X-ray diffraction intensities . The electron density and diffraction intensities are represented by mathematical functions, which may be interconverted variously using the Fourier transform , convolution and complex product operations if the phases of the diffracted X-rays are known. Because the phases are not directly measurable, the dilemma known as the “phase problem” arises. Several methods exist that enable phase estimation and...
This is a preview of subscription content, log in via an institution to check access.
Access this chapter
Subscribe and save.
- Get 10 units per month
- Download Article/Chapter or eBook
- 1 Unit = 1 Article or 1 Chapter
- Cancel anytime
- Available as PDF
- Read on any device
- Instant download
- Own it forever
- Durable hardcover edition
- Dispatched in 3 to 5 business days
- Free shipping worldwide - see info
Tax calculation will be finalised at checkout
Purchases are for personal use only
Institutional subscriptions
Drenth J (1994) Principles of Protein X-ray Crystallography. Springer-Verlag, New York
Google Scholar
Rhodes G (2000) Crystallography Made Crystal Clear, 2nd edn. Academic Press
Download references
Author information
Authors and affiliations.
Max Planck Institute of Biochemistry, Martinsried, Germany
Richard A. Engh
You can also search for this author in PubMed Google Scholar
Corresponding author
Correspondence to Richard A. Engh .
Rights and permissions
Reprints and permissions
Copyright information
© 2006 Springer-Verlag
About this entry
Cite this entry.
Engh, R.A. (2006). X-Ray Crystallography, Basic Principles. In: Encyclopedic Reference of Genomics and Proteomics in Molecular Medicine. Springer, Berlin, Heidelberg . https://doi.org/10.1007/3-540-29623-9_5050
Download citation
DOI : https://doi.org/10.1007/3-540-29623-9_5050
Publisher Name : Springer, Berlin, Heidelberg
Print ISBN : 978-3-540-44244-8
Online ISBN : 978-3-540-29623-2
eBook Packages : Biomedical and Life Sciences Reference Module Biomedical and Life Sciences
Share this entry
Anyone you share the following link with will be able to read this content:
Sorry, a shareable link is not currently available for this article.
Provided by the Springer Nature SharedIt content-sharing initiative
- Publish with us
Policies and ethics
- Find a journal
- Track your research
What is X-ray Crystallography?
- Download PDF Copy
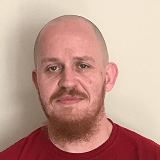
X-Ray crystallography is a tool used to provide structural information about molecules. The technique was developed in 1912 by William Henry Bragg and William Lawrence Bragg (a father and son team who won the 1915 Nobel Prize in Physics for their work in the field), who built upon earlier work by Max von Laue.
Von Laue discovered that by shining X-rays through a copper sulfate crystal onto a photographic plate, diffraction spots that related to the crystalline structure of the sample were produced.
- An introduction to X-ray Crystallography
Methodology
Applications of x-ray crystallography.
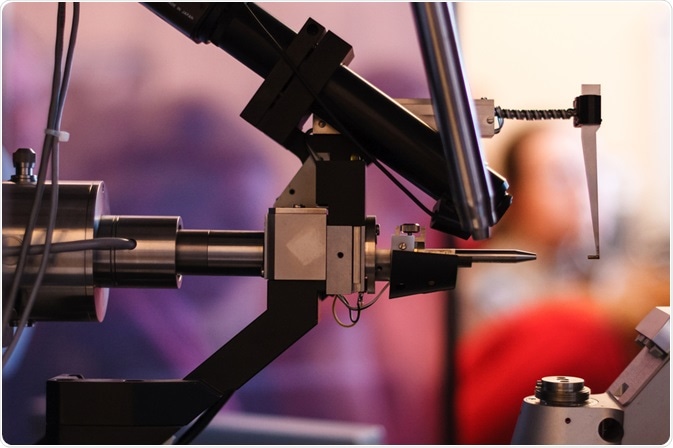
An introduction to X-ray crystallography
X-ray crystallography uses electromagnetic radiation (specifically, X-rays) to determine the molecular and atomic structure of a crystal. The structure of the crystal causes the X-rays to diffract in specific directions. Through analysis of the intensities and angles of these beams, the position and arrangement of electrons within the crystalline structure can be determined.
A three-dimensional picture of the electron densities can then be produced. Information such as the mean position of atoms within the structure, covalent bonding between them, and their crystallographic disorder can then be determined, which represents the three-dimensional structure of a molecule.
The reason X-rays are used in this process is because the clouds of electrons are at the same scale as the X-ray radiation wavelength. This means that the radiation is deflected and scattered by the electrons of the atoms in the crystal. The deflected X-ray beams produce a scattering distribution which is proportional to the scattering angle. Bragg’s law is used to describe this.
Since many diverse types of structure can form crystals, X-ray crystallography can have many research applications. The substances that can be analyzed by this method include salts, minerals, metals, semiconductors as well as biological compounds including proteins, nucleic acids, and vitamins.
The most difficult part of the process is growing a perfect crystal, as this is needed to provide accurate information about the sample. Some macromolecules, especially those with a high atomic weight such as membrane proteins, can be difficult to crystallize.
Many different fields of study including biology, chemistry, and geology have found uses for this powerful yet simple technique.
An X-ray crystallography machine works by utilizing a four-circle diffractometer. This works by rotating the crystal and the deflector between the X-ray source and the screen. The screen receives the X-rays which have passed through the crystal.
X-Ray crystallography experiments are broken down into four steps:
- Protein crystallization
- Production of a diffraction pattern
- Analysis of the diffraction pattern to produce an electron density map
- Determination of the protein structure.
As has been mentioned, the most difficult part of the process is achieving a perfect crystal structure for analysis. As the position of electrons must be mapped accurately, it is important that the structure be flawless.
A diffraction pattern is formed on the screen by the X-rays which have been absorbed by the atoms in the crystal, which leave behind dark diffraction spots. Their density varies with the amount of interference between the diffracted electrons at each point. These spots represent accurately the electron density, which can be mapped.
Once the electron density map has been made, the analysis of the crystallographic data is relatively straightforward. It does, however, require complex mathematics to make sense of the information. In the early days of X-ray crystallography, these calculations were done by hand, but now computers are used to perform them.
The calculation used is called the Fourier transformation. This calculation transforms the data into a three-dimensional representation of the atomic or molecular structure of the sample molecule or material.
X-ray crystallography is used to analyze many different molecules and has been used in many famous projects in the fields of organic and inorganic chemistry. Early structures which were resolved using the technique were simple crystals, including quartz and salt.
One of the most well-known of these was the determination of the double-helical structure of DNA by Franklin, Watson, and Crick in 1953. Other important molecules whose structures were identified include Vitamin B12, insulin, and penicillin.
As well as the analysis of organic molecules (proteins, vitamins, nucleic acids) and inorganic molecules and structures, X-ray crystallography has been used to develop novel materials in both material and life sciences.
X-ray crystallography is still one of the best methods for the structural analysis of many substances. It remains a powerful, simple, and reliable technique that is used by laboratories across the world.
There are numerous studies that X-ray crystallography can provide unique insights for that other methodologies cannot, but it does have some disadvantages compared to these other techniques, which is why it should always be used as part of a suite of analytical methods.
- Smyth, MS, and Martin, JHJ (2000) X-ray crystallography. Molecular Pathology https://www.ncbi.nlm.nih.gov/pmc/articles/PMC1186895/
- Blundell, TL and Johnson, LN (1976). Protein crystallography. London: Academic Press
Further Reading
- All Crystallography Content
- Protein Crystallization
- Protein Structure Determination
- Microseeding Explained
- Protein Crystallization Screening
Last Updated: Oct 14, 2019
Reginald Davey
Reg Davey is a freelance copywriter and editor based in Nottingham in the United Kingdom. Writing for AZoNetwork represents the coming together of various interests and fields he has been interested and involved in over the years, including Microbiology, Biomedical Sciences, and Environmental Science.
Please use one of the following formats to cite this article in your essay, paper or report:
Davey, Reginald. (2019, October 14). What is X-ray Crystallography?. News-Medical. Retrieved on September 23, 2024 from https://www.news-medical.net/life-sciences/What-is-X-ray-Crystallography.aspx.
Davey, Reginald. "What is X-ray Crystallography?". News-Medical . 23 September 2024. <https://www.news-medical.net/life-sciences/What-is-X-ray-Crystallography.aspx>.
Davey, Reginald. "What is X-ray Crystallography?". News-Medical. https://www.news-medical.net/life-sciences/What-is-X-ray-Crystallography.aspx. (accessed September 23, 2024).
Davey, Reginald. 2019. What is X-ray Crystallography? . News-Medical, viewed 23 September 2024, https://www.news-medical.net/life-sciences/What-is-X-ray-Crystallography.aspx.

This is not the best way for introducing XRD. The neccessary basics are all jumped. The useful x-ray wave length is 0.5-2.5 Å which is different from that given in the text. Concluding, the writer speak to a very narrow circle of colleagues.
Cancel reply to comment
- Trending Stories
- Latest Interviews
- Top Life Sciences Articles

How can microdialysis benefit drug development
Ilona Vuist
In this interview, discover how Charles River uses the power of microdialysis for drug development as well as CNS therapeutics.

Global and Local Efforts to Take Action Against Hepatitis
Lindsey Hiebert and James Amugsi
In this interview, we explore global and local efforts to combat viral hepatitis with Lindsey Hiebert, Deputy Director of the Coalition for Global Hepatitis Elimination (CGHE), and James Amugsi, a Mandela Washington Fellow and Physician Assistant at Sandema Hospital in Ghana. Together, they provide valuable insights into the challenges, successes, and the importance of partnerships in the fight against hepatitis.

Addressing Important Cardiac Biology Questions with Shotgun Top-Down Proteomics
In this interview conducted at Pittcon 2024, we spoke to Professor John Yates about capturing cardiomyocyte cell-to-cell heterogeneity via shotgun top-down proteomics.

Latest Life Science News

Newsletters you may be interested in

Your AI Powered Scientific Assistant
Hi, I'm Azthena, you can trust me to find commercial scientific answers from News-Medical.net.
A few things you need to know before we start. Please read and accept to continue.
- Use of “Azthena” is subject to the terms and conditions of use as set out by OpenAI .
- Content provided on any AZoNetwork sites are subject to the site Terms & Conditions and Privacy Policy .
- Large Language Models can make mistakes. Consider checking important information.
Great. Ask your question.
Azthena may occasionally provide inaccurate responses. Read the full terms .
While we only use edited and approved content for Azthena answers, it may on occasions provide incorrect responses. Please confirm any data provided with the related suppliers or authors. We do not provide medical advice, if you search for medical information you must always consult a medical professional before acting on any information provided.
Your questions, but not your email details will be shared with OpenAI and retained for 30 days in accordance with their privacy principles.
Please do not ask questions that use sensitive or confidential information.
Read the full Terms & Conditions .
Provide Feedback

Publications Home — Over 100 years of publishing excellence
- Book Author Resources
- Submit a Book Proposal
- AMS Rights, Licensing, Permissions
- Open Math Notes
- Frequently Asked Questions
- Member Journals
- Research Journals
- Translation Journals
- Distributed Journals
- Open Access Journals
- Guidelines and Policies
- Journal Author Resources
Librarian Resources
- eBook Collections
- COUNTER Usage Statistics
- My Subscriptions
- Subscription Information
- Licensing Information
Mathematical Reviews/MathSciNet®
- MathSciNet ®
- Reviewer Home
- MathSciNet ® Subscriptions
Membership Home — Welcome to your membership center
Membership choices.
- Join the Society
- Renew your Membership
- Gift a Membership
Individual Membership
- Member Benefits
- Member Directory
- Reciprocating Societies
- Members in Developing Countries
Institutional Membership
- Domestic Institutions
- International Institutions
- Two-Year Institutions
- Graduate Student Chapter Program
Other Member Types
- Corporate Memberships
- Associate Memberships
Meetings & Conferences Home — Engage with colleagues and the latest research
National meetings.
- Joint Mathematics Meetings
- Upcoming JMMs
- Previous JMMs
- Special Lectures
- Professional Enhancement Programs (PEPs)
Sectional Meetings
- Upcoming Sectionals
- Previous Sectionals
- Presenting Papers
- Hosting Sectionals
Other Meetings, Conferences & Workshops
- Mathematics Research Communities
- Education Mini-conference
- International Meetings
- Mathematics Calendar
- Short Courses
- Workshop for Department Chairs and Leaders

Meetings Resources
- Suggest a Speaker
- AMS Meetings Grants
- Submitting Abstracts
- Welcoming Environment Policy
- MathSafe – supporting safe meetings
News & Outreach Home — Explore news, images, posters, and mathematical essays
News from the ams.
- AMS News Releases
- Feature Stories
- Information for Journalists
- In Memory Of
Math Voices
- Feature Column
- Math in the Media
- Column on Teaching and Learning
Explorations
- Recognizing Diverse Mathematicians
- AMS Posters
- Mathematics & Music
- Mathematical Imagery
- Mathematical Moments
Grants & Awards Home — Resources and opport unities to further your mathematical pursuits
- Research & Travel Grants
- Child Care Grants for Meetings
- Young Scholars Program Grants
Honors & Awards
- Prizes & Awards
- Fellows of the AMS
AMS Fellowships
- Stefan Bergman Fellowship
- Birman Fellowship for Women Scholars
- Centennial Fellowship
- Claytor-Gilmer Fellowship
Awards & Recognition
- AMS Prizes & Awards
Learning & Careers Home — Resources to support advanced mathematics teaching and learning
For students.
- High School
- Undergraduate
- Find Graduate Programs
- Research Experience for Undergraduates (REUs)
For Faculty & Leaders
- Department Chairs Workshop
- Column on Teaching & Learning
- Salary & Departmental Data
- Employment Services
- BEGIN Careers
Professional Development
- Mathematical Opportunities Listing
- MathPrograms
Government Relations Home — Advocating for the mathematical sciences
Elevating mathematics in congress.
- Our Mission
- Letters, Statements, & Legislation
- Congressional Briefings
Legislative Priorities
- Federal Issues of Concern
- Federal Budget Process
Get Involved
- Advocacy Resources
- Take Action
DC-Based Fellowships
- Congressional Fellowship
- Mass Media Fellowship
- Catalyzing Advocacy in Science & Engineering (CASE) Fellowship
Giving to the AMS — Your gifts make great things happen for mathematics Make a Gift
What you can support.
- The 2020 Fund
- Next Generation Fund
- JMM Child Care Grants
- MathSciNet for Developing Countries
Create a Legacy
- Make a Tribute Gift
- Create a Permanent Fund
- Establish a Prize, Award or Fellowship
- Bequests and Charitable Estate Planning
Honoring Your Gift
- Donor Stories
- Donor Wall of Honor
- Thomas S. Fiske Society
- AMS Contributors Society
- AMS Gardens
Giving Resources
- AMS Development Committee
- AMS Gift Acceptance Policy
About the AMS — Advancing research. Connecting the mathematics community.
Our organization.
- Executive Staff
- Equity, Diversity, & Inclusion
- Jobs at AMS
- Customer Service
Our Governance
- Board of Trustees
- Executive Committee
Governance Operations
- Calendar of Meetings
- Policy Statements & Guidelines
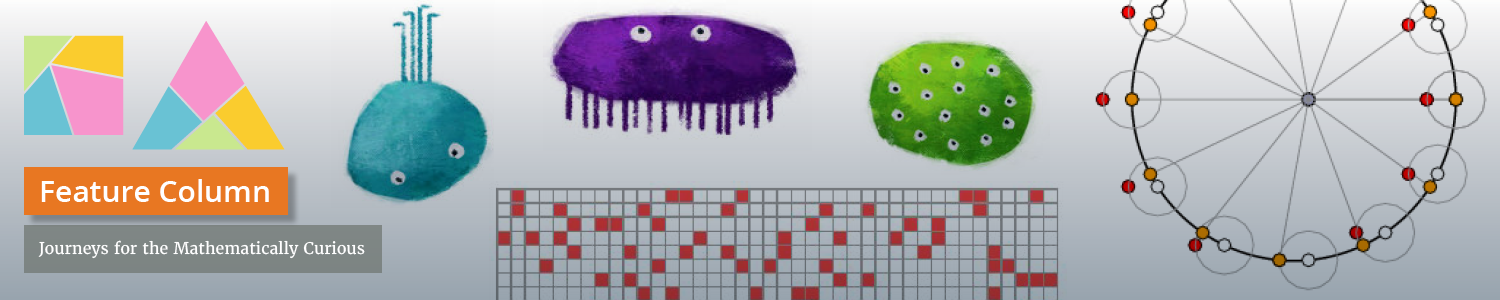
Welcome to the Feature Column
X-ray crystallography and the fourier transform.

Introduction
X-ray crystallography has been essential, since the beginning of the 20th century, to our understanding of matter; recently, as knowledge of the chemical composition of proteins has progressed, the determination of their 3-dimensional structure has become indispensable for the correct interpretation of their functions. Our main access to this information is X-ray crystallography . (X-rays are used because their wavelengths are on the order of inter-atomic distances in molecules, in the range 1-100 Å ; one Å is $10^{-10}$m). Mathematics enters into the process at two stages. The study of space groups tells what periodic configurations are possible in three-space; in fact these are often called "crystallographic groups" by mathematicians. The other connection, the subject of this column, is the surprising and pleasing fact that when a monochomatic X-ray diffracts off a crystal it performs part of a mathematical operation: the Fourier transform (developed in the 19th century in completely different contexts); when the incidence angle is varied, the complete transform is produced. The flaw in this lovely picture is that we cannot measure all the details of the diffracted wave; otherwise the entire molecular structure could be calculated by inverting the Fourier transform. There are many online resources devoted to X-ray crystallography; I have especially profited from Randy Read's Protein Crystallography Course and from Kevin Cowtan's Interactive Structure Factor Tutorial . Thanks also to my Stony Brook colleagues Peter Stephens and Miguel Garcia-Diaz for helpful correspondence and remarks.
Fourier series: temperature distribution in a wire
Fourier series and the Fourier transform were invented as a method of data analysis. For example, let us follow Jean-Baptiste Joseph Fourier (1768-1830) in studying the time evolution of the temperature distribution in a circular loop of circumference $a$, given an initial distribution of temperature $f(x), 0\leq x\leq a$; (we require $f(a)= f(0)$). We start by calculating what are now known as the Fourier coefficients of $f$: $$a_0 = \frac{1}{a}\int_0^af(x)~dx,$$ $$a_h = \frac{2}{a}\int_0^af(x)\cos~ \frac{2\pi h}{a}x~dx,$$ $$b_h = \frac{2}{a}\int_0^af(x)\sin~ \frac{2\pi h}{a}x~dx.$$ It can be proved, if $f$ is sufficiently well-behaved, that the linear combination $$a_0 +\sum_{h=1}^{\infty}a_h\cos~ \frac{2\pi h}{a}x + \sum_{h=1}^{\infty}b_h\sin~ \frac{2\pi h}{a}x$$ (the Fourier series of $f$; when $a=2\pi$ these formulas simplify pleasantly) converges everywhere to $f(x)$.
(Taken as an initial distribution separately, each of the $\cos~ \frac{2\pi h}{a}x$ and $\sin~ \frac{2\pi h}{a}x$ functions determines a simple solution, as does a constant function; the linearity of the heat equation allows these separate solutions to be combined, with coefficients $a_h$ and $b_h$, to give the complete solution to the problem.)
It is useful to simplify the formulas by using Euler's identities $\cos~ t = \frac{1}{2}(e^{it} + e^{-it})$, $\sin~ t = \frac{1}{2i}(e^{it} - e^{-it})$ and grouping terms to yield $$f(x) = \sum_{h=-\infty}^{\infty}c_h e^{i\frac{2\pi h}{a}x}$$ and $$c_h = \frac{1}{a}\int_0^a f(x)~e^{-i\frac{2\pi h}{a}x}~dx;$$ in general, the $c_h$ are complex numbers.
Electron density distribution in a crystal
The dual operations of integration : $f(x)~\rightarrow~ \{c_h\}$ and summation : $\{c_h\}~\rightarrow~f(x)$ can be realized in other contexts. In this column we will consider the function $\rho(x,y,z)$ that gives the electron density distribution in the crystalline state of some compound. Suppose, for simplicity, that the unit building block, corresponding to a molecule of the compound, is a rectangular solid; say with edge-lengths $a, b$ and $c$; these $a$ by $b$ by $c$ solids are stacked in three-space so as to give a structure repeating every $a$ units in the $x$-direction, every $b$ units in the $y$ and every $c$ units in the $z$. Then the function $\rho(x,y,z)$ will be triply periodic, with periods $a, b$ and $c$, and consequently can be represented as a triple Fourier series $$\rho(x,y,z) = \sum_{h=-\infty}^{\infty} \sum_{k=-\infty}^{\infty} \sum_{l=-\infty}^{\infty} c_{hkl}e^{i(\frac{2\pi h}{a}x + \frac{2\pi k}{b}y +\frac{2\pi l}{c}z)},$$ where $$c_{hkl}=\frac{1}{abc}\int_0^a\int_0^b\int_0^c \rho(x,y,z)e^{-i(\frac{2\pi h}{a}x + \frac{2\pi k}{b}y +\frac{2\pi l}{c}z)} ~dx~dy~dz,$$ directly generalizing our formulas for the circle. If the coefficients $c_{hkl}$ are known, the electron density distribution can be calculated, and then the structure of the molecule can be determined. It is therefore remarkable that the diffraction patterns formed when the crystal is bombarded with X-rays contain precious information about the $c_{hkl}$. Roughly speaking, we can imagine the complex numbers $c_{hkl}$ placed at the vertices $(h,k,l)$ of a 3-dimensional lattice; each X-ray diffraction pattern projects this lattice onto the plane of the image plate. If we label by $p_{\Theta}$ the projection produced by a beam meeting the crystal at a generalized angle $\Theta$, a vertex $(h,k,l)$ which is good position with respect to $\Theta$ (this condition also depends on the wavelength $\lambda$ of the radiation) will appear on the plate as a spot at location $p_{\Theta}(h,k,l)$ and of intensity proportional to the square of the absolute value $|c_{hkl}|$. Varying $\Theta$ will bring a new set of vertices into good position; eventually the lattice can be reconstructed, along with the absolute value of the coefficients at the vertices.
The reciprocal lattice
X-rays interact with a crystal through interaction with parallel families of planes. Suppose as before that the unit cell in the crystal is an $a\times b\times c$ rectangular parallelipiped (when $a,b$ and $c$ are all different, this structure is called orthorhombic ). Every triple $(h,k,l)$ of integers defines a family of planes through the crystal, defined by the equation $$\frac{xh}{a}+ \frac{yk}{b}+\frac{zl}{c} = n ~~~~ (n \mbox{ an integer}).$$ Let us change coordinates to $X = \frac{x}{a}$, $Y=\frac{y}{b}$, $Z=\frac{z}{c}$. (We are now in the reciprocal lattice ; for a non-rectangular crystal the change of coordinates is only slightly more complicated). Then the equation defining the planes becomes $$Xh+Yk+Zl=n,$$ for $n$ an integer. For each $(h,k,l)$ this family of parallel planes fills up the crystal, in the sense that each unit cell vertex lies in (exactly) one of them, as is easy to check. We call these the lattice planes . Graphically, it is easier to represent the analogous concept in two dimensions, so we suppress $z$ and $l$ for the moment. Our crystal is then an array of $a\times b$ rectangles; in the $(X,Y)$ coordinates these measure $1\times 1$. The pair $(0,1)$ gives the lines $Y = n$, for $n$ an integer. These lines are parallel to the $X$-axis, and slice through the base of each unit cell. On the other hand, $(1,1)$ gives the lines $X + Y = n$, or $Y = n -X$, $(-2,1)$ gives $Y = n + 2X$, and $(2,-3)$ gives $Y = -n/3 + (2/3)X$. (See image).
X-ray diffraction from a single family of planes
The distance between two planes in the $(h,k,l)$ family is $\frac{1}{\sqrt{h^2+k^2+l^2}}$. In fact, consider the planes $P$ for $n=0$ and $Q$ for $n=1$. The vector $(h,k,l)$ is perpendicular to both planes, so the closest point to $(0,0,0)$ on $Q$ will be on that vector, i.e. of the form $(\alpha h, \alpha k, \alpha l)$ for some number $\alpha$. Since the point is on $Q$, it must satisfy $\alpha h^2+ \alpha k^2 +\alpha l^2 = 1$, so $\alpha = \frac{1}{h^2+k^2+l^2}$, the point is $\frac{1}{h^2+k^2+l^2}(h,k,l)$, and its distance from $(0,0,0)$ is $\frac{1}{\sqrt{h^2+k^2+l^2}}$, as claimed. When a planar beam of wavelength $\lambda$ strikes the crystal, there is possible interference between beams diffracted by electrons in parallel $(h,k,l)$ planes: each family acts like a diffraction grating. The physics of diffraction can be summarized in terms of reflections (even though the signal analyzed is usually transmitted through the crystal). In these terms, a beam reflected from the next plane in will have traveled an extra distance $\ell = 2d\sin\theta$ where $d$ is the distance between the planes, and $\theta$ is the angle of incidence (measured from the horizontal). So the two beams will be have a phase difference of $2\pi\frac{\ell}{\lambda}$. In particular, when $d$ and $\theta$ are such that $\frac{\ell}{\lambda}$ is an integer $n$, i.e. $n\lambda = 2d\sin\theta$, the beams will be exactly in phase; this is Bragg's Law , discovered in 1912. -->
X-ray diffraction: how a monochromatic plane wave performs Fourier analysis on the electron density distribution.
The diffraction corresponding to a diffraction vector ${\bf s}$ and a single electron at position ${\bf r}$ multiplies the amplitude of the scattered wave by a phase factor $e^{-2\pi i {\bf r}\cdot{\bf s}}$. If $\rho({\bf r})$ is the electron density function in the crystal, the effect on ${\bf s}$ will sum to $$ F({\bf s}) = \int_{\mbox{crystal}}\rho({\bf r})e^{-2\pi i {\bf r}\cdot{\bf s}}~ d{\bf r}.$$ So the structure factor $F({\bf s})$ appears as the Fourier transform of the electron density function $\rho({\bf r})$. (Straightforward diffraction experiments only measure the (square of) the absolute value of $F({\bf s})$, which shows up as the intensity of the spot corresponding to ${\bf s}$. If the phases were also known, the Fourier transform could be inverted to give an exact picture of the electron density $\rho({\bf r})$).
It is possible to rewrite this integral in terms of the $(h,k,l)$ vectors in reciprocal space. For each such vector ${\bf H}$ we write, using the reciprocal coordinates ${\bf X} = (X,Y,Z)$ $$ F(h,k,l) = \int_{\mbox{unit cell}}\rho({\bf X})e^{-2\pi i {\bf X}\cdot(h,k,l)}~ d{\bf x}.$$ In this case the inverse Fourier transform $$\rho({\bf X})=\int F(h,k,l)e^{2\pi i {\bf X}\cdot(h,k,l)}~ dV$$ where $dV$ is volume in reciprocal space, can be approximated by a Fourier series $$\rho({\bf X})=\sum_{h=-\infty}^\infty~\sum_{k=-\infty}^\infty~\sum_{l=-\infty}^\infty F(h,k,l)e^{2\pi i {\bf X}\cdot(h,k,l)}$$ which can be compared with the Fourier series for $\rho(x,y,z)$ given at the start of this column.
Here is a nice example, from Kevin Cowtan's Interactive Structure Factor Tutorial . The example is 2-dimensional, and shows how rapidly the structure factors, with their phases , converge to the target structure. The target electron density function looks like this:

Cowtan's simulation leads to the approximate Fourier synthesis of the target from just the seven largest structure factors: those corresponding to $(h,k) = (0,1), (1,0), (-1,2), (-2,1), (1,2), (3,-2), (3,1)$. Here is how the synthesis proceeds, step by step, each time adding in the next structure factor. These images are from his tutorial , and are used with permission. The unit cell (not orthorhombic!) is outlined in dots.

Welcome to the Feature Column!
These web essays are designed for those who have already discovered the joys of mathematics as well as for those who may be uncomfortable with mathematics. Read more . . .
Search Feature Column
Feature Column at a glance
Source: http://chemwiki.ucdavis.edu/Analytical_Chemistry/Instrumental_Analysis/Diffraction/X-ray_Crystallography
Browse Course Material
Course info.
- Prof. Donald Sadoway
Departments
- Materials Science and Engineering
As Taught In
- Chemical Engineering
Learning Resource Types
Introduction to solid state chemistry, 15. introduction to crystallography.
« Previous | Next »
Session Overview
Electronic Materials, Crystalline Materials | |
p-n junction, introduction to the solid state, the 7 crystal systems, the 14 Bravais lattices, properties of cubic crystals: simple cubic, face-centered cubic, body-centered cubic, and diamond cubic | |
subvalent, aliovalent, supervalent, conduction band, valence band, semiconductor, silicon, dopant, thermal excitation, n-type, p-type, acceptor level, charge carrier, p-n junction crystal, glass, amorphous solid, ordered solid, long-range order, Bravais lattice, crystal system, point group, translation, rotation, symmetry plane, degree of symmetry, crystal basis, unit cell, face-centered cubic, simple cubic, body-centered cubic, hexagonal close-packed, rock salt structure, diamond cubic, birefringence, crystallography, nearest neighbor, Auguste Bravais, René Haüy, Robert Hooke, Christiaan Huygens, Nicolaus Steno | |
silicon (Si), boron (B), diamond (C) glass, obsidian, quartz, calcite, tin (Sn), basalt, beryl, fluorite, gold (Au), aluminum (Al), copper (Cu), platinum (Pt), methane ice (CH ), rock salt (NaCl) | |
transistors, diodes, current rectification cannonball stacking, tiling of 2D surfaces, fiber optics coupling, optical beam-splitter, colored gold |
Prerequisites
Before starting this session, you should be familiar with:
- Semiconductor properties and behavior ( Session 14 )
- Basic geometry in 2D and 3D
- Bond angles and lengths in molecules ( Session 11 )
Looking Ahead
This session introduces the cubic unit cells, a key framework for discussing atomic-level processes in solids throughout this module and in later topics, such as Diffusion ( Session 24 ) and Solid Solutions ( Session 33 onwards). The next module on Amorphous Solids ( Session 21 onwards) discusses non-crystalline materials in more detail, contrasting their structure and properties with the ordered solids studied here.
Learning Objectives
After completing this session, you should be able to:
- Classify materials as n- or p-type , and explain how simple p-n junction devices work.
- Derive the 7 crystal systems by varying the lattice constants a, b, c and angles α, β, γ.
- For a given repeating pattern, determine the crystal basis and Bravais lattice .
- Sketch the simple cubic , body-centered cubic , and face-centered cubic structures, and calculate key parameters such as the lattice constant , atomic radius , and packing density .
Archived Lecture Notes #4 (PDF) , Sections 1-3
Book Chapters | Topics |
---|---|
12.1, “Crystalline and Amorphous Solids.” | Crystal lattice parameters; properties of crystalline and amorphous solids |
12.2, “The Arrangement of Atoms in Crystalline Solids.” | The unit cell; packing of spheres |
3.1, “Seven Systems and Fourteen Lattices.” | The unit cell and its parameters; crystal systems and crystal (Bravais) lattices |
3.2, “Metal Structures.” | Body-centered cubic, face-centered cubic/cubic close-packed, and hexagonal close-packed structures; atomic packing factor; plane stacking |
Lecture Video
- Download video
- Download transcript
Lecture Slides (PDF - 3.2MB)
Lecture Summary
Continuing last lecture’s explanation of extrinsic semiconductors , the Electronic Materials module ends at 13:00 with an exploration of p-type doping and an overview of the p-n junction . Prof. Sadoway moves on to introduce a classification for materials based on the degree of atomic-level order , contrasting ordered solids ( crystals , e.g. quartz, calcite) with amorphous solids ( glasses , e.g. obsidian). The 7 crystal systems and 14 Bravais lattices are introduced:
- Tetragonal (e.g. tin (Sn), basalt)
- Hexagonal (e.g. beryl)
- Rhombohedral (e.g. calcite)
- Cubic (e.g. fluorite, gold (Au), aluminum (Al), copper (Cu), platinum (Pt), methane (CH 4(s) ), rock salt (NaCl))
- Orthorhombic
Crystal structures are described using a basis , which may be an atom, a group of ions (e.g. rock salt (NaCl)), or a molecule (e.g. methane (CH 4(s) ), proteins), repeated at the points of a Bravais lattice. Since they apply to many common metals and minerals, this course focuses on the cubic crystal systems : simple, body-centered, and face-centered.
Problems (PDF)
Solutions (PDF)
Textbook Problems
[Saylor] Sections | Conceptual | Numerical |
---|---|---|
12.2, “The Arrangement of Atoms in Crystalline Solids.” | 1, 8, 9 | 3, 5, 9, 11 |
12.3, “Structures of Simple Binary Compounds.” | 4 | none |
For Further Study
Supplemental readings.
Hooke, Robert. Micrographia; or, Some Physiological Descriptions of Minute Bodies Made by Magnifying Glasses, with Observations and Inquiries . London, England: J. Martyn and J. Allestry, 1665. [ View on Project Gutenberg ]
Chapman, Allan. England’s Leonardo: Robert Hooke and the Seventeenth-Century Scientific Revolution . Philadelphia, PA: Institute of Physics Publishing, 2005. ISBN: 9780750309875.
Steno, Nicolaus. The Prodromus of Nicolaus Steno’s Dissertation Concerning a Solid Body Enclosed by Process of Nature within a Solid . Translated by John Garrett Winter. New York, NY: Macmillan, 1916.
Cutler, Alan. The Seashell on the Mountaintop: A Story of Science, Sainthood, and the Humble Genius Who Discovered a New History of the Earth . New York, NY: Plume, 2004. ISBN: 9780452285460.
Dijksterhuis, Fokko Jan. Lenses and Waves: Christiaan Huygens and the Mathematical Science of Optics in the Seventeenth Century . Boston, MA: Kluwer, 2004. ISBN: 9789048167067.
Auguste Bravais
René Just Haüy
Robert Hooke
Christiaan Huygens
Niels Steensen (Nicolaus Steno)
M. C. Escher
Georges Braque
Vallier, Dora. Braque: The Complete Graphics: Catalogue Raisonne . New York, NY: Alpine Fine Arts Collection, 1988. ISBN: 9780881680065.
Talking Heads. “Burning Down the House.” Speaking in Tongues . Sire Records, 1983.
Other OCW and OER Content
Content | Provider | Level | Notes |
---|---|---|---|
DoITPoMS | Undergraduate | ||
Connexions | Undergraduate | ||
MIT OpenCourseWare | Graduate | A mathematical approach to crystal symmetry with connections to bulk material properties such as stress, strain, thermal conductivity, and piezoelectricity. |

You are leaving MIT OpenCourseWare

An official website of the United States government
The .gov means it’s official. Federal government websites often end in .gov or .mil. Before sharing sensitive information, make sure you’re on a federal government site.
The site is secure. The https:// ensures that you are connecting to the official website and that any information you provide is encrypted and transmitted securely.
- Publications
- Account settings
The PMC website is updating on October 15, 2024. Learn More or Try it out now .
- Advanced Search
- Journal List
- Acta Crystallogr F Struct Biol Commun
- v.73(Pt 4); 2017 Apr 1

Cryo-electron microscopy and X-ray crystallography: complementary approaches to structural biology and drug discovery 1
Catherine vénien-bryan.
a Institut de Minéralogie, de Physique des Matériaux et de Cosmochimie, UMR 7590 CNRS, UPMC, IRD, MNHN, 4 Place Jussieu, 75005 Paris, France
Laurent Vuillard
b Chimie des Protéines, Pôle d’Expertise Biotechnologie, Chimie, Biologie, Institut de Recherches Servier, 125 Chemin de Ronde, 78290 Croissy-sur-Seine, France
Jean Albert Boutin
c Pôle d’Expertise Biotechnologie, Chimie, Biologie, Institut de Recherches Servier, 125 Chemin de Ronde, 78290 Croissy-sur-Seine, France
Associated Data
Supplementary Table S1, some of the most exciting cryo-EM studies that can be found in the PDB.. DOI: 10.1107/S2053230X17003740/hv5346sup1.pdf
Single-particle cryo-electron microscopy (cryo-EM) has gained its ‘ lettres de noblesse ’ in structural biology. The synergistic convergence of technological and computational advances now make this a feasible method for determining structures at near-atomic to atomic resolution (∼5–2 Å). The potential impact of cryo-EM on drug discovery is discussed, together with how the method can provide information on conformational variability connected to the function of macromolecular complexes.
The invention of the electron microscope has greatly enhanced the view scientists have of small structural details. Since its implementation, this technology has undergone considerable evolution and the resolution that can be obtained for biological objects has been extended. In addition, the latest generation of cryo-electron microscopes equipped with direct electron detectors and software for the automated collection of images, in combination with the use of advanced image-analysis methods, has dramatically improved the performance of this technique in terms of resolution. While calculating a sub-10 Å resolution structure was an accomplishment less than a decade ago, it is now common to generate structures at sub-5 Å resolution and even better. It is becoming possible to relatively quickly obtain high-resolution structures of biological molecules, in particular large ones (>500 kDa) which, in some cases, have resisted more conventional methods such as X-ray crystallography or nuclear magnetic resonance (NMR). Such newly resolved structures may, for the first time, shed light on the precise mechanisms that are essential for cellular physiological processes. The ability to attain atomic resolution may support the development of new drugs that target these proteins, allowing medicinal chemists to understand the intimacy of the relationship between their molecules and targets. In addition, recent developments in cryo-electron microscopy combined with image analysis can provide unique information on the conformational variability of macromolecular complexes. Conformational flexibility of macromolecular complexes can be investigated using cryo-electron microscopy and multiconformation reconstruction methods. However, the biochemical quality of the sample remains the major bottleneck to routine cryo-electron microscopy-based determination of structures at very high resolution.
1. Introduction
Biochemists focus on processes that occur at the molecular level and are therefore invariably fascinated by the small structural details. For instance, visualizing the interaction between a molecule (natural or synthetic) and its protein target has been like the quest for the holy grail for many scientists in the search for enzyme inhibitors. In this context, the drug-discovery process became ‘easier’ and more rational with the complementary use of co-crystallization or ‘soaking’ methods combined with structural biology studies and medicinal chemistry. In this case, co-crystallization applies to the targeted protein and active substances. Note also that active substances are often improperly called ‘drugs’, whereas they are in fact substances that are far from having the characteristics that will admit them to the pharmacopoeia. Nowadays, the active molecule docked in its target can be visualized, either in a solid-state interaction (thanks to co-crystallization or soaking methods and the use of these co-crystals in X-ray diffraction) or in some rare cases in a more dynamic interaction (NMR) with their targets. These techniques have provided medicinal chemists with concrete and highly informative data on the interaction between a molecule and its target protein. The medicinal chemists then orchestrate the synthetic steps leading first to active substances and then to drugs.
Even though crystallography has represented a major advance in drug discovery, the process is still complex. Indeed, a succession of critical and unpredictable steps make it possible to obtain the structures of target proteins: the construction of a plasmid encoding the targets, often excluding overly flexible parts (identified by prediction); the expression of these proteins in efficient expression systems such as bacteria, yeast or insect cells; purification to homogeneity; the identification of crystallization conditions (which are often strictly dependent on the protein sequence); recording the X-ray diffraction data (often on a synchrotron beamline); processing the data and generating electron-density maps; and interpretation of the maps to produce a three-dimensional model of the protein (see, for example, Friedmann et al. , 2011 ▸ ). The aim is to see and understand the structural details that will allow the investigator to build a model showing interactions between different proteins, between parts of the same protein, or between a compound and a protein.
Until the last two decades or so, to aim at the discovery of an optimal inhibitor of an enzyme in the context of drug discovery, successive molecules (obtained from synthesis and derived from some starting candidate) were each analysed using functional tests which provide an understanding of the structure–activity relationship within the chemical series. There is no doubt that modern drug-discovery programmes are now based on the capacity to either measure the molecule/protein target interactions using biophysical methods (see Renaud et al. , 2016 ▸ ) and/or to visualize at the molecular level the position of the compound on its protein target (see reviews by Zuercher et al. , 2016 ▸ ; Brader et al. , 2017 ▸ ). The latter is essentially based on progress in protein crystallization, co-crystallization approaches and crystal X-ray diffraction analyses. These processes have been reviewed at length. They represent an immense step in understanding molecular pharmacology at the closest level, and have made it possible for medicinal chemists to gain information leading them to rationally synthesize series of molecules designed to become drug candidates. Such successful examples are scattered throughout the medicinal chemistry literature and can be found easily (see, for example, Huang et al. , 2016 ▸ ; Gustafsson et al. , 2017 ▸ ; Hazel et al. , 2017 ▸ ; Mills-Davies et al. , 2017 ▸ ).
Why, then, be jubilant at the recent performance of cryo-electron microscopy (cryo-EM)? Let us remember that the data that have been collected and that populate the PDB (Protein Data Bank; http://www.rcsb.org/pdb ) were mostly obtained by X-ray crystallography from rigid structures. This rigid state shows the interactions between atoms only in the most stable state, which is not necessarily the most common or the most representative state in a cell. Remember also that what is shown under crystallographic conditions is a protein. This is of course an accomplishment in itself, even if it has become routine to obtain such visualizations of new targets. In recent years, complementary techniques to crystallography have emerged. They are interesting because they reveal proteins in states that are not (or are less) rigid, and are more likely to be those of the proteins in solution. Indeed, cryo-EM is particularly well suited for obtaining structural information on large protein complexes and for systems that exhibit multiple conformational or compositional states. In this respect, there is a groundswell of enthusiasm for this approach (Rupp, 2015 ▸ ; Vinothkumar, 2015 ▸ ). We had already sensed this shift several years ago (Jonic & Vénien-Bryan, 2009 ▸ ). It is certainly no coincidence that the January 2016 issue of Nature Methods designated single-particle cryo-electron microscopy technology the ‘Technology of the Year’ (Eisenstein, 2016 ▸ ). In the meantime, discussion of the role of cryo-EM in drug discovery as a complement to protein crystallography continues (Merino & Raunser, 2017 ▸ ; Orlov et al. , 2016 ▸ ; Takizawa et al. , 2017 ▸ ; Wang & Wang, 2017 ▸ ). Of particular interest, Merk and coworkers have presented data demonstrating that cryo-EM can be used to investigate a broad spectrum of drug–target interactions and of dynamic conformational states (Merk et al. , 2016 ▸ ).
2. What is cryo-electron microscopy?
A number of excellent reviews of electron microscopy are available (Nagayama, 2011 ▸ ; Oxley et al. , 2017 ▸ ; Schroder, 2015 ▸ ). The purpose here is not to recapitulate in detail the nature of and the progress in this field, but to highlight recent developments that have been reported. The history of electron microscopy and its application to the observation of biological objects has been marked by major discoveries, each of which has helped to push the resolution limit. These findings relate to sample preparation, microscope performance, image capture or processing of these images. Transmission electron microscopy is an ideal tool for investigating biological specimens, even if at present they are held in a vacuum chamber because electrons do not travel very far in air. These conditions do not yet allow an adequate exploration of the structure of living cells. Spectacular observations of biological material ‘live’ in the electron microscope using a sample holder equipped with microfluidic technologies have been described (Peckys et al. , 2015 ▸ ), but this is not the subject of this commentary. A major advance in electron microscopy was made in the late 1980s, when Jacques Dubochet and coworkers used liquid ethane for the sample preparation of proteins, viruses and macromolecules (Dubochet et al. , 1988 ▸ ). Ultra-rapid freezing to temperatures below −185°C was, and remains, essential for good sample preparation. Indeed, the cooling rate is sufficiently fast to prevent water crystallizing, and rather promotes the formation of vitreous ice (Dubochet et al. , 1988 ▸ ). The sample embedded in vitreous ice is free of stains and artefacts associated with them (typical stains are heavy-metal salts such as uranyl acetate). The sample is then placed in the vacuum chamber of the electron microscope and observed at −180°C. This spectacular progress in sample preparation turned electron microscopy into cryo-EM. For thirty years now, this method of preparation of biological samples has been a major tool in structural biology for the observation of objects in an environment that is less artificial than the solid state observed in a crystal, which was a prerequisite for any high-resolution study. In these fast-freezing conditions, the sample is surrounded by ice in a vitreous state. The density of this vitreous ice is lower than that of proteins or nucleic acids. This difference in density is essential to identify the single particles in the images. In addition, the fast-freezing technique preserves the characteristics of biological structures at atomic resolution and the low temperature provides some protection against the damage induced by electron bombardment. Images are captured during the observation with an electron microscope operating at 200 or 300 kV, and analyses are subsequently performed to reveal the structure of the macromolecules. The projected images of the biological macromolecules originate from multiple random orientations in suspension in the vitreous film. The three-dimensional structure is then calculated by combining and reconstructing projection images into a three-dimensional volume (Fig. 1 ▸ , left). Because the information from each image is noisy and incomplete, hundreds of thousands of imaged particles must be aligned and averaged. The quality of the final reconstructed map of the macromolecule thus depends on the accuracy with which the orientations of the particles are determined relative to each other; this is performed using ad hoc software. Over the last forty years, the group of Joachim Frank has developed methods for the image analysis of isolated particles (Frank, 2006 ▸ ). They demonstrated that by combining images from many particles and identifying the relative orientations of these particles on the electron-microscopy grid, they could increase the signal-to-noise ratio, allowing the calculation of structures in three dimensions. This method is more efficient for large particles because these are more easily visible. There is therefore a lower size limit for the visualization of macromolecules in cryo-EM. Until very recently, it was around 250 kDa. However, it is claimed that in theory, starting from perfect images, one could obtain a final resolution of a reconstruction volume of 3 Å from only 12 000 particles as small as 40 kDa (Henderson, 1995 ▸ ). Despite the dramatic advances in cryo-EM, until a few years ago the resolution of biological structures was mostly limited to 6 or 8 Å and, at this stage, did not compete with data determined by X-ray crystallography or NMR (Ménétret et al. , 2007 ▸ ; Myasnikov et al. , 2005 ▸ ; Vénien-Bryan et al. , 2009 ▸ ).

Schematic representation of three-dimensional single-particle reconstruction. The high purity of the sample is important, as in X-ray crystallography. Initially, the observation of the sample in negative staining is a useful step; as it clearly displays the sample, homogeneity can be checked. The particles are selected from the micrographs, centred and aligned. The classification and averaging images can improve the signal-to-noise ratio, and the class averages can be used to calculate an initial model at low resolution. This is calculated using the common-lines method or the random conical tilt series method (Dubochet et al. , 1988 ▸ ). Then, in the cryo-EM step, the vitrified sample is imaged using a direct electron detector (a film is obtained from multiple images of the same field of the electron-microscope grid). Correcting for movement between the various images of the film is performed. The particles are then windowed and averaged. Determination of the defocus value allows correction of the contrast-transfer function (CTF). After alignment and classification of the data set, the orientation of each projected particle relative to the initial model is assigned. Refinement of the orientation values is performed iteratively until the three-dimensional structure of the macromolecule of interest converges (this figure was adapted from Boutin et al. , 2016 ▸ ).
3. Cryo-electron microscopy and its unique challenges
The two main problems that hinder the determination of biological structures at high resolution using cryo-EM combined with image analysis are (i) the low signal-to-noise ratio (or low contrast) of the captured images, which limits the size of the macromolecules observed (ideally these should be larger than 250 kDa) and (ii) electron beam-induced movement during exposure, which results in degradation of the image quality. The main challenge in cryo-EM is the low signal-to-noise ratio or, in other words, the poor contrast of the image. This low contrast is an inevitable consequence of the low atomic mass of the elements that constitute biological objects (proteins, nucleic acids, carbohydrates, lipids). Exposures above a dose limit of electrons irreversibly break the covalent bonds, resulting in destruction of the very details that we seek to elucidate. Therefore, very low doses of electrons are used when biological structures are observed in cryo-EM. Another difficulty that can thwart attempts to obtain high-resolution information is inherent to the interaction of electrons with the sample. Indeed, in order to obtain detailed biological structural information, the particles must not move more than one ångström, equivalent to the diameter of an H atom. However, more extensive motions appear when the electron beam interacts with a biological sample. This is owing to several things. Firstly, the impact of the electrons on the vitreous ice film in which the biological particles are embedded may cause thermal expansion and the release of constraints at the level of this film. In addition, electron-beam irradiation can break the covalent bonds of biological structures, generating molecules in the gaseous state (such as hydrogen, oxygen, nitrogen and methane; Henderson, 1995 ▸ ), which escape from the vitreous ice film. These sample movements are inevitably induced by the electron beam and have severely hampered the collection of high-resolution images in the past. Recording an image in low-dose conditions using a conventional medium (film photography or CCD cameras) takes a few seconds, during which the movement of the biological object will degrade the high-resolution data.
4. Why a revolution? Recent technological and methodological innovations
Since 2012, new direct electron detectors (DEDs) have become available. In the past, electron-microscope images were recorded on silver photographic film and then digitized. The advent of image acquisition from CCD (charge-coupled device) detectors thus allowed automated data collection, leading to an increase in the number of images and the size of a data set. However, there is a major limitation for CCD cameras. Incident electrons initially interact with a particular scintillator, which converts them into photons that are then detected. The electron-scattering events in the scintillator give rise to a large ‘cloud’ of photons and electrons, each contributing to the formation of the image. The size of this cloud is larger than the size of the CCD camera pixels; indeed, it can be spread over many pixels. The result is an attenuation of high-resolution signals and thus a limitation of the final resolution.
The new direct electron CMOS (complementary metal-oxide semiconductor) detector overcomes the scintillator step of CCD cameras. A number of technical obstacles had to be overcome to make these new cameras available, the most important of which was the development of a radiation-resistant support to reduce deterioration of the silicon chip. These cameras display a remarkable sensitivity that can significantly increase the image contrast. The easy viewing of ‘small’ proteins of about 100 kDa is now possible. In addition, structural information at high frequencies is preserved. Another remarkable quality of these new DEDs is their fast readout rate: instead of recording one image per second (as for movies and CCD cameras), these new cameras record some movies at a rate of 24–40 frames per second. Thus, we have access to successive images of the same field just like in a video film and the high-speed readout allows the detection of very small movements (on the ångström scale) in these images. The movements of the particles are then corrected from one frame to the other using appropriate software. Each video image is then aligned and stacked to give a final image that is much sharper as each particle is aligned from one frame to the next (Fig. 2 ▸ ).

Motion compensation and recovery of high-resolution information. Averaging of successive frames from a movie of phosphorylase kinase (a 1.3 MDa macromolecule) taken from the same area on the electron-microscope grid before ( a ) and after ( b ) alignment. The structural features of the protein are blurred before alignment.
In parallel to the marketing of the new DED cameras, improved image-processing software has emerged: firstly, movie-processing software to perform the correction or the compensation of movements between frames described above (Campbell et al. , 2012 ▸ ; Li et al. , 2013 ▸ ) and, secondly, software for the automated collection of images and software to extract detailed structural information from images captured in an optimal way. For instance, incorporation of the maximum-likelihood approach in three-dimensional calculation has made it possible to determine multiple structures at high resolution of a dynamic structure using one cryo-EM data set (Lyumkis et al. , 2013 ▸ ; Scheres & Chen, 2012 ▸ ). The maximum-likelihood (ML) method was introduced by Sigworth (1998 ▸ ). It is a supervised classification method which is based on the Bayes theorem. It makes use of a discriminant function to assign a pixel to the class with the highest likelihood. In other words, it does not give a specific direction to a particle with reference to a similarity test, but rather a set of probabilities of being in a certain direction. Such methods have proven their ability to handle noisy data. Thus, the programs RELION (Scheres & Chen, 2012 ▸ ) and FREALIGN (Lyumkis et al. , 2013 ▸ ) are now widely used. RELION , with its user-friendly interface, operates mainly without operator intervention, calculates less noisy models in three dimensions and provides quality-control criteria and validation of the final structure with objective criteria and therefore produces more reliable results (Rosenthal & Henderson, 2003 ▸ ; Scheres & Chen, 2012 ▸ ; Fig. 1 ▸ ). Moreover, this software has a powerful classification method that is particularly effective for sorting particles in more homogeneous structural subclasses, which allow the separation of the different conformational states of molecules that are simultaneously present in a preparation deposited on the electron microscope.
5. Recent advances in computational methods for analyzing the conformational variability of macromolecular complexes from cryo-electron microscopy images
In cryo-EM the samples can display different conformational states. Whilst such heterogeneity can limit the resolution of a three-dimensional reconstruction, the identification of distinct states permits investigators to model dynamics and conformational transitions, thereby providing information on biological function. A striking example is that of the AMPA receptor, for which Dürr et al. (2014 ▸ ) made a film showing large conformational rearrangements of the N-terminal domain and of the ligand binding to the desensitized state. Other noteworthy examples have recently been published, including an analysis of transcribing ribosomes (Abeyrathne et al. , 2016 ▸ ; Behrmann et al. , 2015 ▸ ), understanding the conformational changes necessary for the function of hexameric ATPase p97 (Banerjee et al. , 2016 ▸ ) and a view of the proton-translocation-driven rotational fluctuations of the mitochondrial ATP synthase (Zhou et al. , 2015 ▸ ). In principle, heterogeneous samples (from a biochemical point of view) can also be classified using the same method. Here, the sample may be purified to some extent by image processing rather than during the tedious steps of biochemical purification, but we are still far from this vision.
Conformational variability analysis from cryo-EM images is usually performed by classifying the images into a number of discrete classes representing all of the conformational states present in the specimen. However, recent methods take continuous conformational changes into account (Jonić, 2017 ▸ ).
Discrete-state methods based on conformational variability analysis in three dimensions can be classified into the following three groups: multi-reference classification, ML classification and classification based on statistical analysis. Supervised multi-reference classification approaches require a good prior knowledge of all conformational states that coexist in the sample (Gao et al. , 2004 ▸ ). ML methods estimate a set of density maps that best describe the given heterogeneous set of images (Lyumkis et al. , 2013 ▸ ; Scheres & Chen, 2012 ▸ ). Classification based on statistical analysis does not require prior knowledge of conformers, but relies on a preliminary model to assign orientation and translation parameters to each image (Klaholz, 2015 ▸ ; Penczek et al. , 2011 ▸ ).
Discrete-state classification methods usually contribute to high-resolution reconstructions of single conformations. Within the high-resolution reconstruction strategies, classification methods are used to select a subset of images containing the particles with the most consistent views and conformations ( i.e. only those assigned to the classes of highest resolution), and the three-dimensional reconstruction is then computed using only this image subset. In this context, many particle images are thrown away without fully understanding the reasons for their inconsistency with other particles. For instance, a three-dimensional reconstruction at near-atomic resolution is sometimes obtained by collecting 0.5–1.0 million particle images, but retaining only one half after a two-dimensional classification and less than one quarter or even 10% for the final three-dimensional reconstruction (Merk et al. , 2016 ▸ ; Walls et al. , 2016 ▸ ).
However, the classes of lower resolution could come from a mixture of particles with different (possibly unique) conformations that are difficult to represent with the given, usually small, number of classes. Discrete-state methods may provide a limited view of data heterogeneity because of the use of a small number of classes. Continuous-state methods can provide a broader view of data heterogeneity than discrete-state methods (Jonić, 2017 ▸ ).
While discrete-state methods assume that a few distinct conformational states coexist in the sample, continuous-state methods assume a continuum of conformational states. Currently, only two approaches consider continuous conformational changes explicitly. These are usually referred to as HEMNMA (hybrid electron-microscopy normal-mode analysis; Jin et al. , 2014 ▸ ) and manifold embedding (Dashti et al. , 2014 ▸ ). In the HEMNMA method, the conformational variability is directly analysed and visualized in three-dimensional space. The method analyses EM images using the normal modes of a starting three-dimensional model obtained by EM or X-ray crystallography. The manifold-embedding approach analyses the conformational variability in two-dimensional space determined by one projection direction (using images with virtually the same orientations) and then combines the conformational variability information obtained in different projection directions to visualize it in three dimensions. It is assumed that the effects of orientation changes dominate those of conformational changes. This method does not require knowledge of the number of states/classes.
6. Major breakthroughs: some examples
The first structures at near-atomic resolution were obtained for large complexes with high symmetry, such as viruses with icosahedral symmetry (Yu et al. , 2008 ▸ ; Zhang et al. , 2008 ▸ ). Several studies have recently shown that cryo-EM can be used to obtain near-atomic resolution structures of small complexes (170–150 kDa) with low symmetry (Bartesaghi et al. , 2015 ▸ ; Liao et al. , 2013 ▸ ; Walls et al. , 2016 ▸ ) or no symmetry (Bai et al. , 2015 ▸ ; Wan et al. , 2016 ▸ ), where the best resolution (1.8 Å) was obtained for the 334 kDa glutamate dehydrogenase (Merk et al. , 2016 ▸ ), in which small-molecule inhibitors were localized and the conformational changes induced by the binding of an allosteric inhibitor were defined.
Over the last few years, the number of published structures studied using the methods described above, with a similar structural quality to those obtained by X-ray crystallography, has shown exponential growth. In Supplementary Table S1 we list some of the most exciting cryo-EM studies that can be found in the PDB. With in excess of 30 000 structures since the beginning of 2010, we had to limit our query to proteins and objects with a molecular weight of below 500 kDa. Therefore, this table gives a flavour of the explosion of structures that have been deposited in recent years. Indeed, there are proteins for which several dozen structures have been deposited in the presence of various compounds: substrates and inhibitors, for example. We provide a single entry per protein, complex, particle or (subcellular) structure. It is obvious that those 127 entries represent a huge range of biological structures, some of which were calculated with an outstanding overall resolution ( i.e. below 5 Å).
We have also plotted the year of deposition versus the resolution of the structures (Fig. 3 ▸ ). This figure shows a large increase in high-resolution data from 2012–2013 (with the best resolution being 2.8 Å), but it is also worth pointing out that a fair number of structures at a resolution better than 10 Å were published before 2012, i.e. before the recent advances.

Representation of the evolution of the cryo-EM resolution as a function of time. The data extracted from the PDB (see Supplementary Table S1 for structures obtained using cryo-EM data) since 2010 were plotted against the corresponding resolution. 16 754 entries (the number of structures) were used to build this figure. Many were similar proteins or structures in different conditions or in the presence of different compounds. The table was constructed as an extract of this initial figure comprising a single entry per deposition.
7. The remarkable case of the ryanodine receptor RyR1
The structure of ryanodine receptor type 1 (RyR1), a large endoplasmic macromolecule of 4 × 500 000 Da, has been determined at 3.8, 6.1 and 4.8 Å resolution (Efremov et al. , 2015 ▸ ; Yan et al. , 2015 ▸ ; Zalk et al. , 2015 ▸ ). This macromolecule regulates the massive outflow of calcium from the endoplasmic reticulum of skeletal or cardiac muscle, which initiates contraction. There are two ryanodine receptor subtypes: RyR1 and RyR2. The former is mainly expressed in skeletal muscle and the latter in the heart. The sequence of the RyR2 gene spans 790 000 base pairs and comprises 105 exons (Otsu et al. , 1990 ▸ ). From a sequence point of view, these two receptors are similar, with 66% amino-acid identity. They are similar enough, at least, for specialists in this area to choose to study RyR1 rather than RyR2, which is a far more relevant target from a pathological prospect but has eluded purification for over a decade (including in the hands of our own group). RyR1 is easier to purify than RyR2, which is the reason why these studies were conducted on RyR1 receptors, the structure of which may also help in understanding the functions of RyR2. This channel is tightly regulated by a series of regulatory proteins, including the proline isomerase calstabin. Until recently, the structures of RyR1 and RyR2 were only partially known (Tung et al. , 2010 ▸ ), making it difficult to fully understand the mechanism of action and regulation at the molecular level. In addition, this lack of structural information complicates the synthesis of new regulatory molecules other than ryanodine and its analogues (Sutko et al. , 1997 ▸ ), the peptide imperatoxine (El-Hayek et al. , 1995 ▸ ) or rapamycin derivatives (Brillantes et al. , 1994 ▸ ). An X-ray crystallographic approach was not appropriate for such a large assembly. The protein from skeletal muscle (non-recombinant) can be purified to high homogeneity using affinity chromatography. The three-dimensional structure of this huge complex (2 × 10 6 Da) was determined using a relatively low number of particles: 100 000, 230 000 and 245 000 particles, respectively, from 500 to 3400 micrographs with a resolution from 3.8 to 6.1 Å (Efremov et al. , 2015 ▸ ; Yan et al. , 2015 ▸ ; Zalk et al. , 2015 ▸ ). The crystallographic structures of many different domains or subunits of the complex were then positioned in the three-dimensional envelope(s) calculated from the cryo-EM data. This demonstrates the complementarity between cryo-EM and X-ray diffraction in the analyses of complex macromolecules (Wang & Wang, 2017 ▸ ). Determining the architecture of the multi-protein complex allows a better understanding of the interactions between the different parts and defines the site where some pharmacological regulators interact. The large number of mutations in RyR2 (expressed in the heart; Brini, 2004 ▸ ) and their association with heart pathologies makes this receptor a target for anti-arrhythmic drug development. It is therefore vital to have access to the detailed structure of this complicated molecular machine in order to elaborate the pharmacological pathways of regulation (Fig. 4 ▸ ), even though the limitations of these approaches for these particular receptors have already been pointed out (Yuchi & Van Petegem, 2016 ▸ ).

( a ) Structure of ryanodine receptor type 1 (RyR1) from Efremov et al. (2015 ▸ ). The resolution is 4.8 Å. (i) The molecular architecture of RyR1 determined by cryo-electron microscopy. (ii) Schematic representation of the middle section in the plane of the channel: dark blue, N-terminal domain; cyan, Spry domains 1, 2 and 3; pink, ‘repeat domains’ 1, 2, 3 and 4; green, solenoid connecting domain; red, central solenoid domain; orange, transmembrane and C-terminal domains. Calstabine is coloured yellow. Global representations from cryo-EM perpendicular to the membrane (iii) and views parallel to the membrane from above (iv) and from below (v) are shown. The same colour coding is used as above. ( b ) Schematic representation of ryanodine receptor type 1. The diagram in ( a ) is taken from the structures obtained by crystallography and X-ray diffraction. They were placed on the three-dimensional envelope obtained by cryo-electron microscopy. The PDB codes are 5c30 for tandem domains 1 and 2; 2xoa for the N-terminal domain AB part with the two solenoids A; 4p9j for Spry 2; 5c33 for Spry 1; 4ert for tandem domains 3 and 4; and 1qrq for the β-subunit of the voltage-gated potassium channel. This figure was adapted from Boutin et al. (2016 ▸ ).
Other examples, such as work on complexes such as the nuclear pore (Stuwe et al. , 2015 ▸ ), provide new information on these structures that is in most cases inaccessible to the crystallographer because they are too large and complex.
8. Zooming in on cellular processes at the molecular level using cryo-electron tomography
The methodological developments described above also benefit cellular ultrastructure imaging using cryo-electron tomography (cryo-ET). The principle of tomography is based on the collection of images from samples tilted at different angles containing a number of copies of the macromolecular complex of interest. If the number of different views (projections) of the biological object is sufficient, three-dimensional information on the initial volume can be calculated. This step is called tomographic reconstruction. Subvolumes of the macromolecule of interest are then cut, extracted from the tomogram, aligned and sorted (the subtomogram averaging method). Averaging the different copies of the present structure in different directions can improve the resolution. Thus, a large number of homogeneous subvolumes facilitates the analysis and can provide the molecular characteristics at medium resolution. Therefore, cryo-ET has the capability to obtain nanometre-scale (medium-resolution) information about macromolecular complexes in their native environment, and thus provides a bridge between light microscopy and in vitro structure-determination methods. This is important because many complexes cannot be purified. Knowing both the structure and the location of macromolecular complexes is crucial for understanding their cellular functions. The nuclear periphery of a HeLa cell has been investigated using these methods (Mahamid et al. , 2016 ▸ ). Assisted by the synergistic application of recent technical developments, cryo-ET holds promise for revealing the molecular organization giving rise to cellular function in unperturbed environments. These invaluable methods of investigation in vivo could be used to test new compounds directly on targeted proteins in their natural environment.
9. Perspectives
Although recent developments in cryo-EM have been revolutionary, the development of new pharmacological inhibitors requires structural data at better than 3 Å resolution. Such a resolution currently remains inaccessible for all but a small set of targets. But for how long? The method continues to evolve and improvements are ongoing. Among these are the following.
- (i) The development of cameras with improved direct detection of electrons, with thinner sensor chips and with a faster readout rate, together improving the signal-to-noise ratio at high resolution.
- (ii) The improvement of the ‘phase plate’ technology. These thin-film phase plates consist of a continuous amorphous carbon film of appropriate thickness with a small central hole placed in the back focal plane of the objective lens of the electron microscope. The zero-order beam passes through the central hole while scattered electrons are phase-shifted by −π/2, resulting in a threefold to fivefold increase in specimen contrast. This will be advantageous for the study of small proteins.
- (iii) The motion-compensation methods currently used are still relatively poorly effective for the first frames of the movie pictures. It is precisely at this time that the movements are the largest, but the structural information at the highest resolution disappears quickly because of damage owing to irradiation (Scheres & Chen, 2012 ▸ ). Solving this problem is crucial and will provide information before the onset of irreparable radiation phenomena.
- (iv) Improvements in sample preparation, for example the use of materials that are less likely to move under the electron beam or methods for the easier visualization of small proteins such as the use of specific antibodies (especially Fab fragments) or of fusion proteins in order to more effectively identify proteins on the microscope grid. Such molecules can be used not only as ‘benchmarks’ for better alignment during the classification of objects, but can also help to validate a reconstruction (Wu et al. , 2016 ▸ ). The laboratory of Holger Stark has introduced two techniques to improve complex stability and homogeneity. These techniques are called GraFix (Kastner et al. , 2008 ▸ ) and ProteoPlex (Chari et al. , 2015 ▸ ). GraFix improves both the compositional and structural homogeneity of the samples. ProtoPlex is a high-throughput method that systematically tests the stability of a complex in various buffer conditions. The approach uses a dye that fluoresces in the polar environment created when proteins unfold. This allows the rapid screening of buffer conditions that improve sample quality. The method also accommodates the addition of small molecules, inhibitors, agonistic ligands or antibodies to directly screen for their ability to alter conformational stability.
The immediate challenges that we face are the high costs of the purchase and maintenance of the powerful microscopes that are required to elucidate high-resolution structures. The computation-time requirements of the image-processing pipeline are also important and require supercomputers. Poor access to these instruments (in relation to the growing demand) and their costs call for coordinated funding models and group use. Also, correct sample preparation, image collection and analysis is not as easy as it sounds and appropriate training is required.
Rather than imaging technologies or image-processing methods, we contend that the major bottleneck to routine cryo-EM determination of structures at resolution close to 2 Å is currently the preparation of specimens of adequate quality that take into account intrinsic protein flexibility. There are reasons to think that once these new developments in equipment and software have been implemented in a practical way, maybe in the not-too-distant future, the dream of using cryo-EM to determine protein structures at atomic resolution using only a few thousand particles, as described by Henderson (1995 ▸ ), will finally become a reality.
10. Conclusion
It is clear to us that the arsenal of methods available to obtain structural data from proteins increases the range of possibilities. It is also clear that, contrary to Callaway’s statement that ‘the revolution will not be crystallized’ (Callaway, 2015 ▸ ), the crystallographic approach remains the method of choice, and perhaps the easiest route, to continue to obtain the information necessary for the discovery and development of new chemical molecules for medical use targeting proteins of ‘reasonable’ size. This arsenal obviously also includes attractive methodologies such as cryo-EM. What has not changed, however, is the imperative need for high-quality ( i.e. purified to homogeneity) proteins or objects to be observed, even if, as mentioned previously, image analysis might be taken as an ultimate purification step. Indeed, the process of purification remains key in this context, and the return to favour of methods such as affinity chromatography (as described in Zalk et al. , 2015 ▸ ) remains one of the steps that will help in obtaining better objects to look at, with a chance of enhancing the resolution of the observations.
One would love to think that today we could analyse everything using cryo-EM, but this faces some practical difficulties, not least the purchase of the microscope.
Supplementary Material
Acknowledgments.
CVB and ZL would like to thank UPMC and CNRS for grants.
1 This paper is an extension of Boutin et al. [(2016 ▸ ), Med. Sci. (Paris) , 32 , 758–767].
- Abeyrathne, P. D., Koh, C. S., Grant, T., Grigorieff, N. & Korostelev, A. A. (2016). Elife , 5 , e14874. [ PMC free article ] [ PubMed ]
- Bai, X.-C., Yan, C., Yang, G., Lu, P., Ma, D., Sun, L., Zhou, R., Scheres, S. H. W. & Shi, Y. (2015). Nature (London) , 525 , 212–217. [ PMC free article ] [ PubMed ]
- Banerjee, S. et al. (2016). Science , 351 , 871–875. [ PMC free article ] [ PubMed ]
- Bartesaghi, A., Merk, A., Banerjee, S., Matthies, D., Wu, X., Milne, J. L. & Subramaniam, S. (2015). Science , 348 , 1147–1151. [ PMC free article ] [ PubMed ]
- Behrmann, E., Loerke, J., Budkevich, T. V., Yamamoto, K., Schmidt, A., Penczek, P. A., Vos, M. R., Bürger, J., Mielke, T., Scheerer, P. & Spahn, C. M. (2015). Cell , 161 , 845–857. [ PMC free article ] [ PubMed ]
- Boutin, J. A., Li, Z., Vuillard, L. & Vénien-Bryan, C. (2016). Med. Sci. (Paris) , 32 , 758–767. [ PubMed ]
- Brader, M. L., Baker, E. N., Dunn, M. F., Laue, T. M. & Carpenter, J. F. (2017). J. Pharm. Sci. 106 , 477–494. [ PubMed ]
- Brillantes, A. B., Ondrias, K., Scott, A., Kobrinsky, E., Ondriašová, E., Moschella, M. C., Jayaraman, T., Landers, M., Ehrlich, B. E. & Marks, A. R. (1994). Cell , 77 , 513–523. [ PubMed ]
- Brini, M. (2004). Biochem. Biophys. Res. Commun. 322 , 1245–1255. [ PubMed ]
- Callaway, E. (2015). Nature (London) , 525 , 172–174. [ PubMed ]
- Campbell, M. G., Cheng, A., Brilot, A. F., Moeller, A., Lyumkis, D., Veesler, D., Pan, J., Harrison, S. C., Potter, C. S., Carragher, B. & Grigorieff, N. (2012). Structure , 20 , 1823–1828. [ PMC free article ] [ PubMed ]
- Chari, A. et al. (2015). Nature Methods , 12 , 859–865. [ PMC free article ] [ PubMed ]
- Dashti, A., Schwander, P., Langlois, R., Fung, R., Li, W., Hosseinizadeh, A., Liao, H. Y., Pallesen, J., Sharma, G., Stupina, V. A., Simon, A. E., Dinman, J. D., Frank, J. & Ourmazd, A. (2014). Proc. Natl Acad. Sci. USA , 111 , 17492–17497. [ PMC free article ] [ PubMed ]
- Dubochet, J., Adrian, M., Chang, J. J., Homo, J. C., Lepault, J., McDowall, A. W. & Schultz, P. (1988). Q. Rev. Biophys. 21 , 129–228. [ PubMed ]
- Dürr, K. L., Chen, L., Stein, R. A., De Zorzi, R., Folea, I. M., Walz, T., Mchaourab, H. S. & Gouaux, E. (2014). Cell , 158 , 778–792. [ PMC free article ] [ PubMed ]
- Efremov, R. G., Leitner, A., Aebersold, R. & Raunser, S. (2015). Nature (London) , 517 , 39–43. [ PubMed ]
- Eisenstein, M. (2016). Nature Methods , 13 , 19–22. [ PubMed ]
- El-Hayek, R., Lokuta, A. J., Arévalo, C. & Valdivia, H. H. (1995). J. Biol. Chem. 270 , 28696–28704. [ PubMed ]
- Frank, J. (2006). Three-Dimensional Electron Microscopy of Macromolecular Assemblies. Oxford University Press.
- Friedmann, D., Messick, T. & Marmorstein, R. (2011). Curr. Protoc. Protein Sci. , Unit 17.4. https://doi.org/10.1002/0471140864.ps1704s66. [ PMC free article ] [ PubMed ]
- Gao, H., Valle, M., Ehrenberg, M. & Frank, J. (2004). J. Struct. Biol. 147 , 283–290. [ PubMed ]
- Gustafsson, R., Jemth, A. S., Gustafsson, N. M., Färnegårdh, K., Loseva, O., Wiita, E., Bonagas, N., Dahllund, L., Llona-Minguez, S., Häggblad, M., Henriksson, M., Andersson, Y., Homan, E., Helleday, T. & Stenmark, P. (2017). Cancer Res. 77 , 937–948. [ PubMed ]
- Hazel, P., Kroll, S. H., Bondke, A., Barbazanges, M., Patel, H., Fuchter, M. J., Coombes, R. C., Ali, S., Barrett, A. G. & Freemont, P. S. (2017). ChemMedChem. 12 , 372–380. [ PubMed ]
- Henderson, R. (1995). Q. Rev. Biophys. 28 , 171–193. [ PubMed ]
- Huang, K.-W., Hsu, K.-C., Chu, L.-Y., Yang, J.-M., Yuan, H. S. & Hsiao, Y.-Y. (2016). J. Med. Chem. 59 , 8019–8029. [ PubMed ]
- Jin, Q., Sorzano, C. O., de la Rosa-Trevín, J. M., Bilbao-Castro, J. R., Núñez-Ramírez, R., Llorca, O., Tama, F. & Jonić, S. (2014). Structure , 22 , 496–506. [ PubMed ]
- Jonić, S. (2017). Curr. Opin. Struct. Biol. 43 , 114–121. [ PubMed ]
- Jonic, S. & Vénien-Bryan, C. (2009). Curr. Opin. Pharmacol. 9 , 636–642. [ PubMed ]
- Kastner, B. et al. (2008). Nature Methods , 5 , 53–55. [ PubMed ]
- Klaholz, B. P. (2015). Open J. Stat. 5 , 820–836.
- Li, X., Mooney, P., Zheng, S., Booth, C. R., Braunfeld, M. B., Gubbens, S., Agard, D. A. & Cheng, Y. (2013). Nature Methods , 10 , 584–590. [ PMC free article ] [ PubMed ]
- Liao, M., Cao, E., Julius, D. & Cheng, Y. (2013). Nature (London) , 504 , 107–112. [ PMC free article ] [ PubMed ]
- Lyumkis, D., Brilot, A. F., Theobald, D. L. & Grigorieff, N. (2013). J. Struct. Biol. 183 , 377–388. [ PMC free article ] [ PubMed ]
- Mahamid, J., Pfeffer, S., Schaffer, M., Villa, E., Danev, R., Kuhn Cuellar, L., Forster, F., Hyman, A. A., Plitzko, J. M. & Baumeister, W. (2016). Science , 351 , 969–972. [ PubMed ]
- Ménétret, J. F., Schaletzky, J., Clemons, W. M. Jr, Osborne, A. R., Skånland, S. S., Denison, C., Gygi, S. P., Kirkpatrick, D. S., Park, E., Ludtke, S. J., Rapoport, T. A. & Akey, C. W. (2007). Mol. Cell , 28 , 1083–1092. [ PubMed ]
- Merino, F. & Raunser, S. (2017). Angew. Chem. Int. Ed. Engl. 56 , 2846–2860. [ PubMed ]
- Merk, A., Bartesaghi, A., Banerjee, S., Falconieri, V., Rao, P., Davis, M. I., Pragani, R., Boxer, M. B., Earl, L. A., Milne, J. L. & Subramaniam, S. (2016). Cell , 165 , 1698–1707. [ PMC free article ] [ PubMed ]
- Mills-Davies, N. et al. (2017). Acta Cryst. D 73 , 9–21. [ PubMed ]
- Myasnikov, A. G., Marzi, S., Simonetti, A., Giuliodori, A. M., Gualerzi, C. O., Yusupova, G., Yusupov, M. & Klaholz, B. P. (2005). Nature Struct. Mol. Biol. 12 , 1145–1149. [ PubMed ]
- Nagayama, K. (2011). J. Electron Microsc. (Tokyo) , 60 , S43–S62. [ PubMed ]
- Orlov, I. et al. (2016). Biol. Cell , 109 , 81–93.
- Otsu, K., Willard, H. F., Khanna, V. K., Zorzato, F., Green, N. M. & MacLennan, D. H. (1990). J. Biol. Chem. 265 , 13472–13483. [ PubMed ]
- Oxley, M. P., Lupini, A. R. & Pennycook, S. J. (2017). Rep. Prog. Phys. 80 , 026101. [ PubMed ]
- Peckys, D. B., Korf, U. & de Jonge, N. (2015). Sci. Adv. 1 , e1500165. [ PMC free article ] [ PubMed ]
- Penczek, P. A., Kimmel, M. & Spahn, C. M. (2011). Structure , 19 , 1582–1590. [ PMC free article ] [ PubMed ]
- Renaud, J.-P., Chung, C.-W., Danielson, U. H., Egner, U., Hennig, M., Hubbard, R. E. & Nar, H. (2016). Nature Rev. Drug. Discov. 15 , 679–698. [ PubMed ]
- Rosenthal, P. B. & Henderson, R. (2003). J. Mol. Biol. 333 , 721–745. [ PubMed ]
- Rupp, B. (2015). Trends Biochem. Sci. 40 , 419–421. [ PubMed ]
- Scheres, S. H. W. & Chen, S. (2012). Nature Methods , 9 , 853–854. [ PMC free article ] [ PubMed ]
- Schroder, R. R. (2015). Arch. Biochem. Biophys. 581 , 25–38. [ PubMed ]
- Sigworth, F. J. (1998). J. Struct. Biol. 122 , 328–339. [ PubMed ]
- Stuwe, T., Correia, A. R., Lin, D. H., Paduch, M., Lu, V. T., Kossiakoff, A. A. & Hoelz, A. (2015). Science , 347 , 1148–1152. [ PMC free article ] [ PubMed ]
- Sutko, J. L., Airey, J. A., Welch, W. & Ruest, L. (1997). Pharmacol. Rev. 49 , 53–98. [ PubMed ]
- Takizawa, Y., Binshtein, E., Erwin, A. L., Pyburn, T. M., Mittendorf, K. F. & Ohi, M. D. (2017). Protein Sci. 26 , 69–81. [ PMC free article ] [ PubMed ]
- Tung, C.-C., Lobo, P. A., Kimlicka, L. & Van Petegem, F. (2010). Nature (London) , 468 , 585–588. [ PubMed ]
- Vénien-Bryan, C., Jonic, S., Skamnaki, V., Brown, N., Bischler, N., Oikonomakos, N. G., Boisset, N. & Johnson, L. N. (2009). Structure , 17 , 117–127. [ PMC free article ] [ PubMed ]
- Vinothkumar, K. R. (2015). Curr. Opin. Struct. Biol. 33 , 103–114. [ PMC free article ] [ PubMed ]
- Walls, A. C., Tortorici, M. A., Bosch, B. J., Frenz, B., Rottier, P. J., DiMaio, F., Rey, F. A. & Veesler, D. (2016). Nature (London) , 531 , 114–117. [ PMC free article ] [ PubMed ]
- Wan, R., Yan, C., Bai, R., Huang, G. & Shi, Y. (2016). Science , 353 , 895–904. [ PubMed ]
- Wang, H.-W. & Wang, J.-W. (2017). Protein Sci. 26 , 32–39. [ PMC free article ] [ PubMed ]
- Wu, S. et al. (2016). Structure , 20 , 582–592.
- Yan, Z., Bai, X.-C., Yan, C., Wu, J., Li, Z., Xie, T., Peng, W., Yin, C.-C., Li, X., Scheres, S. H. W., Shi, Y. & Yan, N. (2015). Nature (London) , 517 , 50–55. [ PMC free article ] [ PubMed ]
- Yu, X., Jin, L. & Zhou, Z. H. (2008). Nature (London) , 453 , 415–419. [ PMC free article ] [ PubMed ]
- Yuchi, Z. & Van Petegem, F. (2016). Cell Calcium , 59 , 209–227. [ PubMed ]
- Zalk, R., Clarke, O. B., des Georges, A., Grassucci, R. A., Reiken, S., Mancia, F., Hendrickson, W. A., Frank, J. & Marks, A. R. (2015). Nature (London) , 517 , 44–49. [ PMC free article ] [ PubMed ]
- Zhang, X., Settembre, E., Xu, C., Dormitzer, P. R., Bellamy, R., Harrison, S. C. & Grigorieff, N. (2008). Proc. Natl. Acad. Sci. USA , 105 , 1867–1872. [ PMC free article ] [ PubMed ]
- Zhou, A., Rohou, A., Schep, D. G., Bason, J. V., Montgomery, M. G., Walker, J. E., Grigorieff, N. & Rubinstein, J. L. (2015). Elife , 4 , e10180. [ PMC free article ] [ PubMed ]
- Zuercher, W. J., Elkins, J. M. & Knapp, S. (2016). Cell. Chem. Biol. 23 , 173–182. [ PubMed ]

IMAGES
VIDEO
COMMENTS
A powder X-ray diffractometer in motion. X-ray crystallography is the experimental science of determining the atomic and molecular structure of a crystal, in which the crystalline structure causes a beam of incident X-rays to diffract in specific directions. By measuring the angles and intensities of the X-ray diffraction, a crystallographer can produce a three-dimensional picture of the ...
X-ray Source: X-ray crystallography requires a high-intensity X-ray source, typically an X-ray generator or a synchrotron radiation facility. These sources emit X-rays with a specific wavelength, usually in the range of 0.6 to 2.5 angstroms (Å), which is appropriate for interacting with the crystal lattice.
Abstract. X-ray crystallography is the prime technique for determining the 3D structure of chemical and biochemical compounds, providing the conformation of the molecules in the crystal in addition to their configuration. The use of X-rays with wavelengths around 1 Å means that the positions of individual atoms in the molecule can be defined.
CS/CME/BIOPHYS/BMI 279. ll 2015 Ron Dror X-‐ray crystallography is the most common method for determining three-‐dimensional structures of proteins a. other molecules. It involves making a crystal of the molecule to be imaged (with many copies of that molecule packed in a regular three-‐dimensional grid.
In 1953 Rosalind Franklin used X-ray crystallography to produce images from a crystal of DNA. One of those images, called photograph 51, eventually led James Watson, Francis Crick and Maurice ...
X-ray crystallography reveals the spatial structure of molecules by measuring how they scatter X-ray radiation when arranged in a crystal lattice.Two broad fields may be distinguished: small molecule crystallography deals with a small number of atom positions and typically well-ordered crystals, while macromolecular (usually protein) crystallography determines a much larger number of atomic ...
This page contains materials for the session on crystallographic notation and the discovery of x-rays. It features a 1-hour lecture video, and also presents the prerequisites, learning objectives, reading assignment, lecture slides, homework with solutions, and resources for further study.
X-ray crystallography is a powerful approach to determining the arrangement of atoms within a crystal, in which an X-ray beam strikes a crystal and causes the beam of light to spread into many specific directions. ... The process of converting the reciprocal space-representation of the crystal into an interpretable electron density map is known ...
X-ray crystallography is still one of the best methods for the structural analysis of many substances. It remains a powerful, simple, and reliable technique that is used by laboratories across the ...
The Center for X-Ray Crystallography University of Florida Written by Jürgen Koller, amended by Annaliese Thuijs Run XL refinement cycle again (now XL takes sphere representation of the atoms and calculates ellipsoid representation which is more accurate) X. XP Now you can also see some of the protons in the structure as q's.
Our main access to this information is X-ray crystallography. (X-rays are used because their wavelengths are on the order of inter-atomic distances in molecules, in the range 1-100 Å ; one Å is 10 − 10 m). Mathematics enters into the process at two stages. The study of space groups tells what periodic configurations are possible in three ...
Crystallography Information on crystallographic symmetry and related topics has been codified and published in the International Tables for Crystallography Originally published in 1935, the work has been revised and expanded to include all sorts of topics relevant to X-ray Crystallography We will only concern ourselves with material
Abstract. X-ray crystallography is the most common technique for the determination of three-dimensional crystalline structures at the atomic scale. Since the discovery of the diffraction of X-rays by crystals over one hundred years ago, the technique has developed into an indispensable tool for material scientists and structural biologists ...
X-ray Crystallography is a scientific method used to determine the arrangement of atoms of a crystalline solid in three dimensional space. This technique takes advantage of the interatomic spacing of most crystalline solids by employing them as a diffraction gradient for -x-ray light, which has wavelengths on the order of 1 angstrom (10 8 cm). ...
Resources. Lecture Slides (PDF - 3.2MB) Lecture Summary. Continuing last lecture's explanation of extrinsic semiconductors, the Electronic Materials module ends at 13:00 with an exploration of p-type doping and an overview of the p-n junction.Prof. Sadoway moves on to introduce a classification for materials based on the degree of atomic-level order, contrasting ordered solids (crystals, e.g ...
An additional benefit of this technique is that the phase problem in X-ray crystallography can be easily solved by the molecular replacement method, since most commonly used fusion tags have previously solved structures. ... (PDB code 4JL7). (C) Theoretical representation of crystal contact formation in PDZ scaffold mediated protein ...
Schematic representation of three-dimensional single-particle reconstruction. The high purity of the sample is important, as in X-ray crystallography. Initially, the observation of the sample in negative staining is a useful step; as it clearly displays the sample, homogeneity can be checked.
A new teaching resource comprised of raw X-ray diffraction data sets from crystallography experiments has been compiled. The aim of this resource is to provide a tool with which to plug the teaching gap between crystals and chemical structures present at various levels of education, as well as providing examples for early stage researchers and institutions without the requisite instrumentation ...